3 Earth in Space: the Solar System
This chapter is a pre-review version
Learning Outcomes
By the end of this section you should be able to:
- Describe the origin of the Solar System;
- List and describe the main groups of objects in the Solar System;
- Apply scientific laws to the motion of those objects;
- Explain the flow of energy from Sun to the Earth’s surface, and the energy cycle within the Earth system.
The Earth orbits in the Solar System – a system of objects that are orbiting around a fairly ordinary star, the Sun (though it’s special for the Earth because it’s much closer than any of the other stars). We will look briefly at the various objects in the Solar System. These include the planets, including Earth, smaller objects including dwarf and minor planets, asteroids and meteoroids, and comets. All these orbit around the Sun. There are also satellites, or moons, that orbit around most of the planets, and of course Earth’s Moon, one of the largest of these satellites, is quite important to us, as it controls things like tides in the Earth’s oceans. All of these parts of the Solar System give us information about the origin of the Solar System and therefore tell us about the Earth.
The Solar System has some things in common with an isolated system – one which exchanges neither matter nor energy with its surroundings — but that is certainly an oversimplification, as the Solar System loses energy to interstellar space. However, the Solar System gains very little energy or matter from the rest of the Universe in comparison to the Sun’s output.
Some parts of the Solar System haven’t changed much since it formed a little over four and a half billion years ago, but as we will see in this course, the Earth has changed a lot, and continues to change at the present day. Some pieces of the Solar System give us a window back in time, and help us to understand parts of Earth history, including the present.
Origin of the Solar System
New stars are believed to be forming in many parts of our galaxy, where interstellar clouds of dust and gas, called nebulae, gradually come together, or “condense”. The driving force for this condensation is gravity, the attraction between all masses in the Universe, which acts slowly on the dust and gas in a nebula to pull it together, releasing energy as heat in the process.
In earlier textbooks this process was usually illustrated by artists’ impressions of the “nebular hypothesis” and the Presolar Nebula that gave rise to our Solar System. Thanks to the increasing power of telescopes, both on the Earth’s surface and in orbit, we now have pictures of developing star systems that must look very similar to our Solar System around the time of its formation.
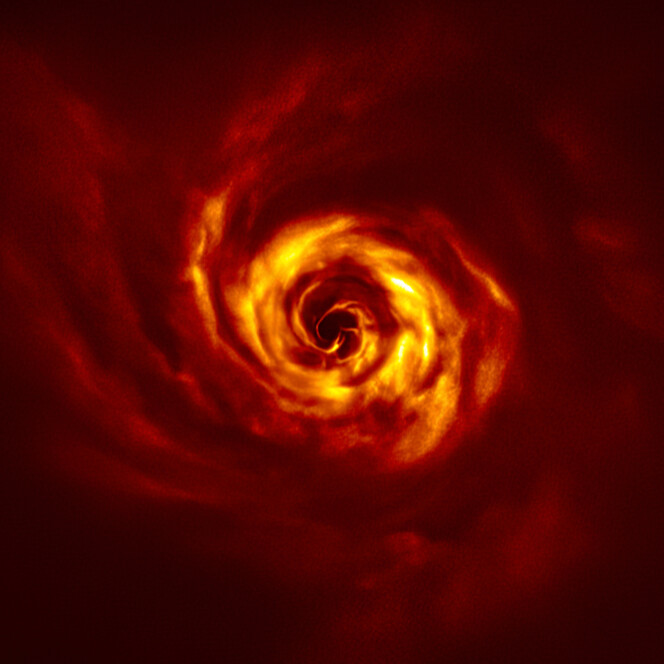
A variety of evidence suggests that the Presolar Nebula, which gave rise to our Solar System. condensed at about 4.6 Ga (Ga is an abbreviation for “Giga-annum” meaning “billions of years ago”). Ages like this are determined using the breakdown of radioactive isotopes, a method that is explained in a later section on the Geosphere. The nebula was undoubtedly rotating as it formed, probably because it inherited some rotation from its parent cloud of dust and gas. We know this because most of the objects in the modern Solar System rotate in the same direction: counterclockwise when viewed from above Earth’s North Pole. As the protoplanetary nebula condensed, the rate of spin became faster because of a principle known as “conservation of angular momentum”: the same phenomenon used by figure skaters performing a spin, who can increase their rotation rate by drawing their arms and legs inward.
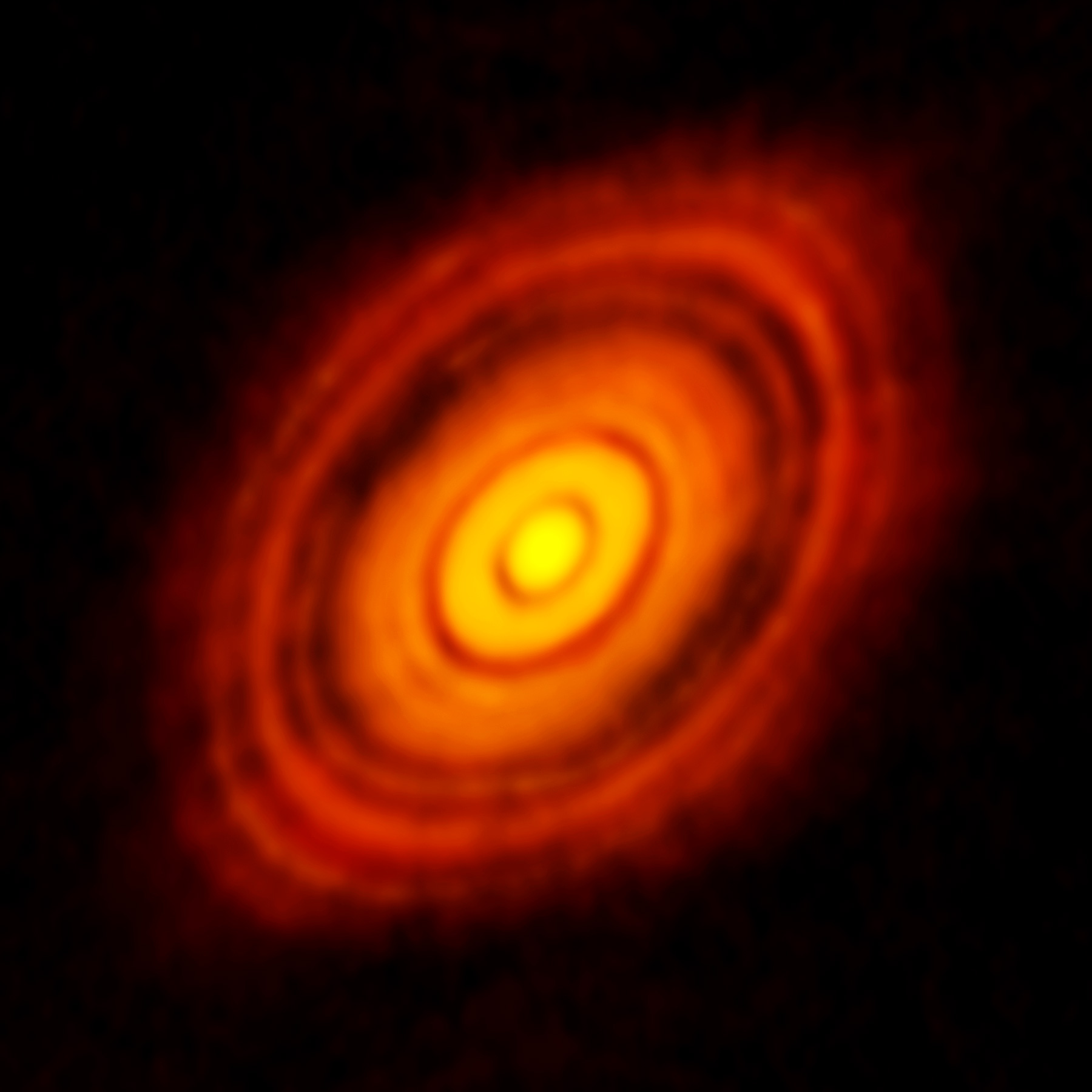
Most of the material collected in the centre, forming the Sun, while the rest became concentrated in a rotating protoplanetary disk. Gravitational attraction within the disk caused the condensation of planets and most of their natural satellites or moons.
The heat released from the collapse of the Sun eventually raised the temperature and pressure to the point where hydrogen, the lightest and most abundant element in the Universe, began to undergo nuclear fusion, producing helium (the same process that occurs in a hydrogen bomb) releasing a much larger, on-going supply of energy that continues to power the Sun at the present day.
Heat from the Sun affected the behaviour of the remaining material in the protoplanetary disk. The lighter elements hydrogen and helium, very abundant in the presolar nebula, and other materials that are gases at moderate temperatures, were driven to the outer parts of the disk, where they ended up in the jovian or outer planets, which are largely made of hydrogen, with only small, rocky cores. Denser elements, like oxygen, iron, magnesium, and silicon remained in the inner part of the disk, that formed the terrestrial or inner planets, which have iron cores surrounded by rocky mantles. The early history of the Solar System was undoubtedly very violent, as objects collided with one another, breaking up and reforming. Some of the effects of bombardment episodes are recorded in planets like Mercury and the Moon, which display huge craters dating back before 3.8 Ga. The Earth was presumably similarly affected, but all traces of these early violent events have been removed by the processes of plate tectonics, weathering, and erosion that have affected the Earth’s much more dynamic surface.
Orbits and gravity
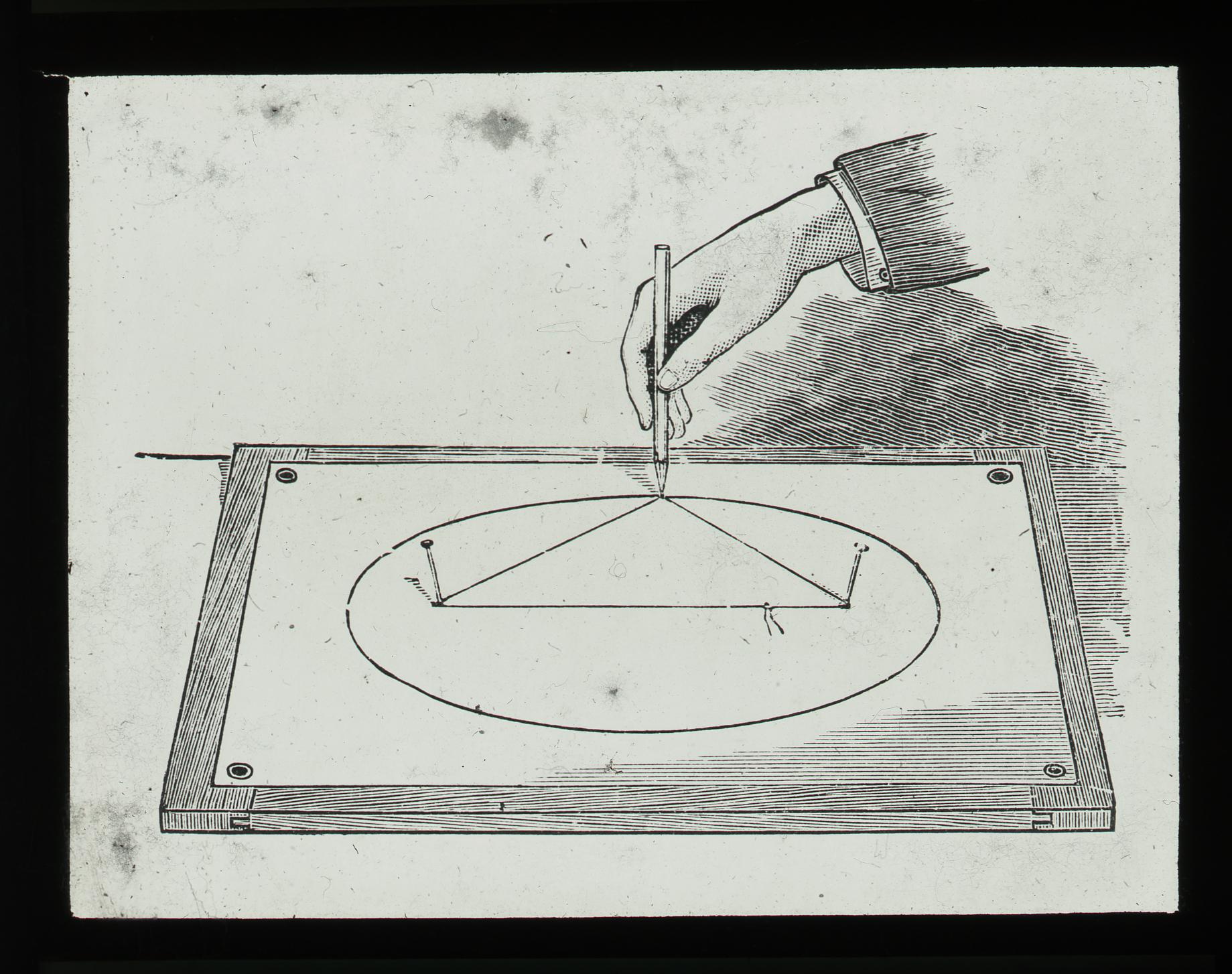
Objects in the Solar System, including Earth, follow orbits that are not exactly circular. Instead they follow an oval path called an ellipse. Mathematically, an ellipse contains two special focus points, or foci. The Sun lies at one focus of the orbit.
This principle was figured out by the German natural philosopher Johannes Kepler around the year 1600 CE, working on observations of the planet Mars, and is known as Kepler’s first law of planetary motion. Kepler also looked at the speeds of Mars in its orbit, and found that the speed varied inversely with distance from the Sun: as Mars got closer to the Sun its speed in its orbit increased. As a result, the planet was said to “sweep out” equal areas in equal times (Kepler’s second law).
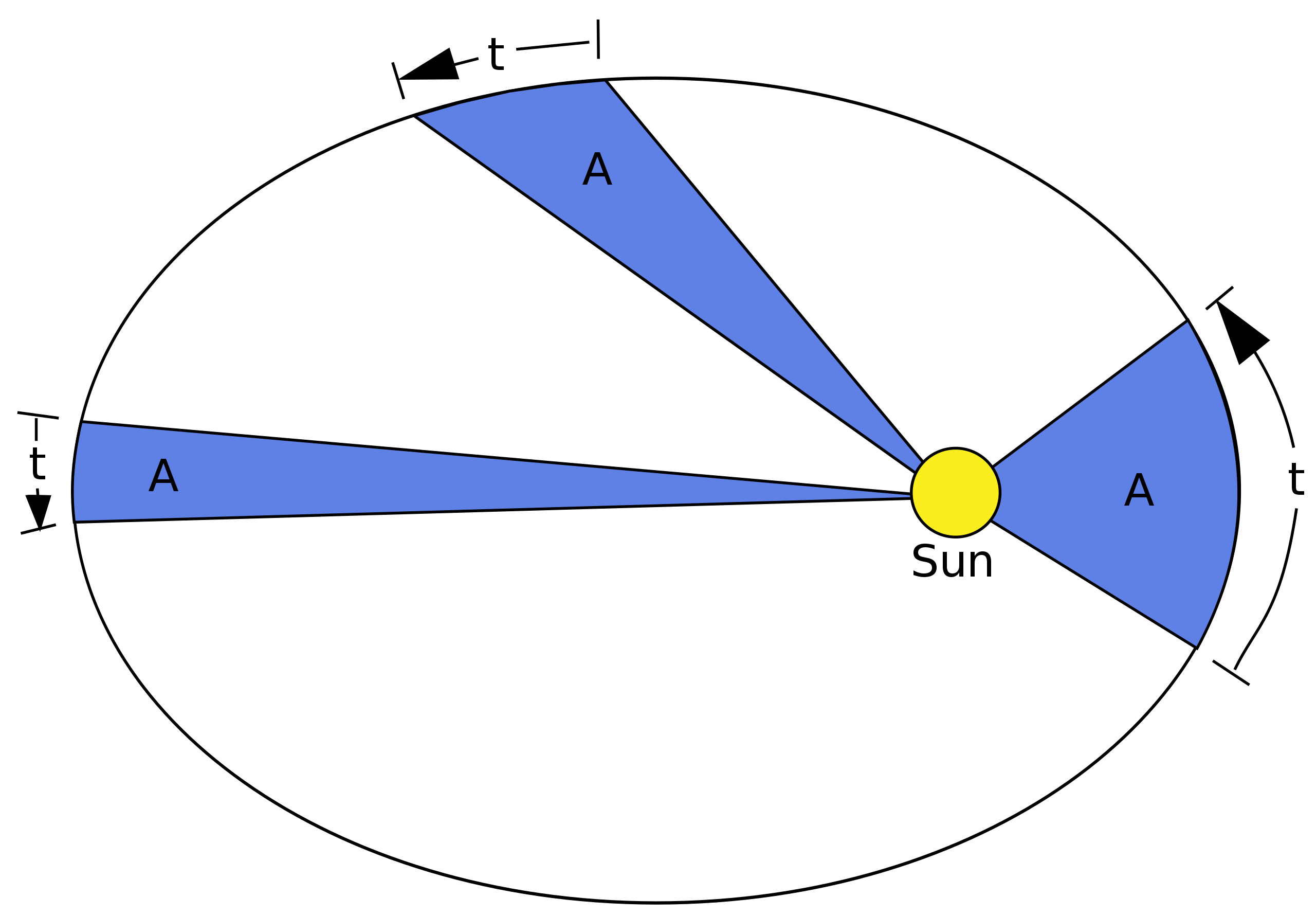
Kepler’s third law dealt with the length of time taken by objects to complete a single orbit around the Sun. He proposed that the square of the orbital period is proportional to the cube of the distance from the Sun. This means that planets in the outer parts of the Solar System take much longer to complete an orbit around the Sun than the Earth (which takes 1 year), while planets with orbits closer to the Sun orbit much faster.
Kepler’s laws were determined empirically: purely by seeking a pattern that explained the observations. Later in the same century, the English natural philosopher Isaac Newton showed that Kepler’s laws can be explained by the force of gravity, a force that acts between all masses in the universe, and which is proportional to the mass of each object and inversely proportional to the square of the distance between them: hence if you move twice as far from the Earth the force of Earth’s gravity declines by a factor of four. Newton also developed the concept of inertia – the idea that a moving object continues in a straight line unless acted on by an outside force (Newton’s first law). Using the laws of gravity and inertia, Newton was able explain all three of Kepler’s empirical laws as a result of physical processes.
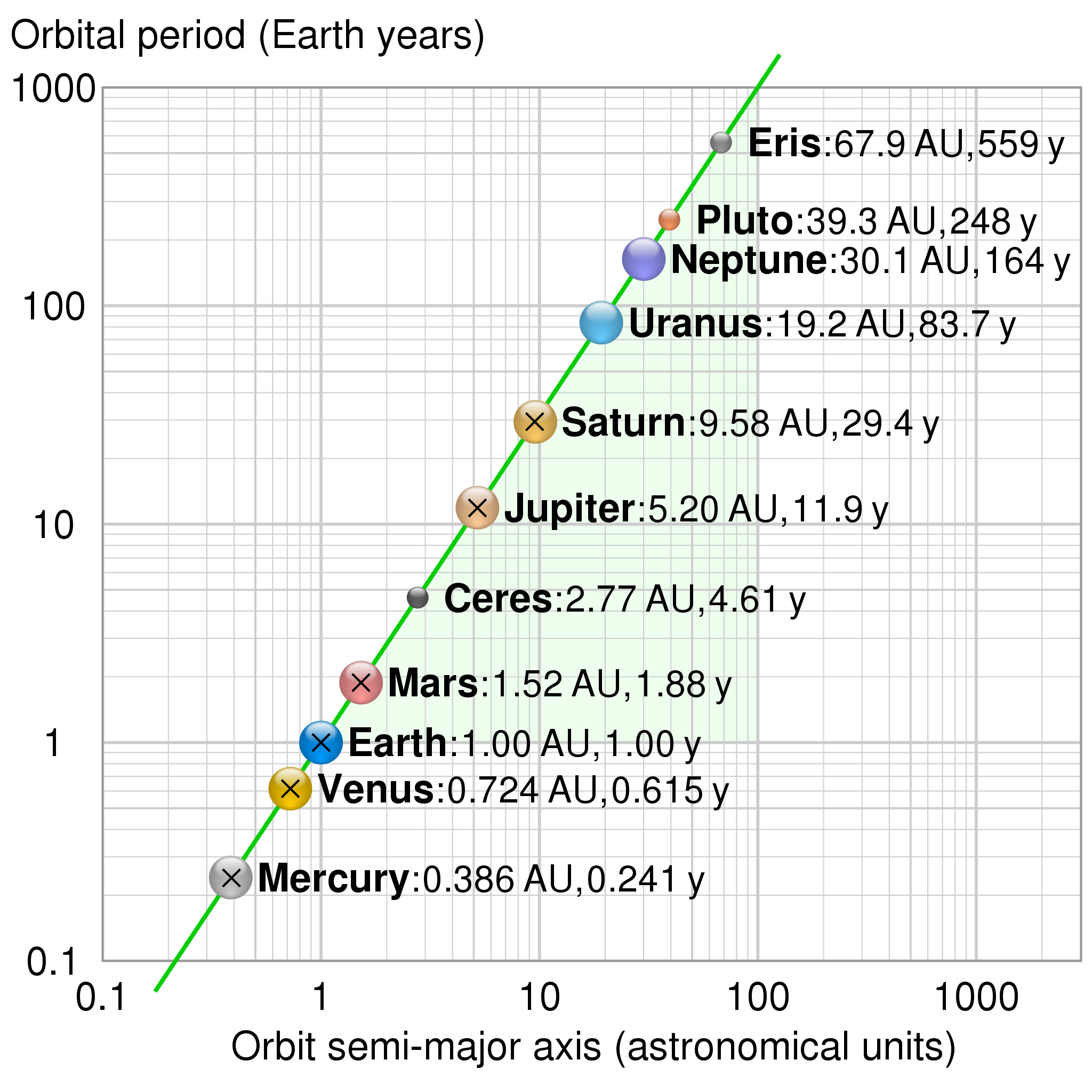
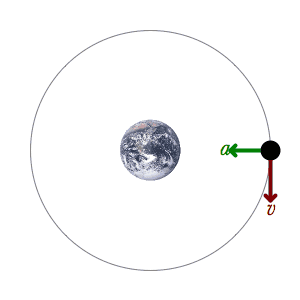
Components of the Solar System
In this section we will take a short tour of the Solar System. By earthbound standards, the Solar System is really large. For example, the average distance from the Earth to the Sun is about 150 million kilometres, or 1.5 x 109 m. To simplify things, this distance is known as 1 astronomical unit.
A graphic simulation of the layout of the Solar System at any moment can be found at
https://theskylive.com/3dsolarsystem
We start with the small objects that most closely resemble samples of the presolar nebula, left over from the origin of the Solar System at about 4.6 Ga. Because of the temperature structure of the protoplanetary disk, these objects are mostly found far away from the Sun. Then we will work inwards towards the more familiar objects near the centre, like the Earth and the Sun.
Comets
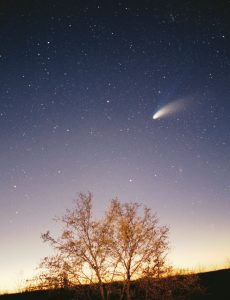
Comets are small but fascinating bodies that mostly orbit very far out beyond the orbit of the planet Neptune, over 30 astronomical units from the Sun. However, they tend to follow highly eccentric elliptical orbits that can bring them close to the Sun and the Earth. (Eccentric orbits are long thin ellipses in which the largest diameter is very much greater than the smallest diameter.) Because they formed at the outer edges of the Solar System they may have compositions that are probably close to the original composition of the protoplanetary disk, and they have a lot of volatile material, substances that we would describe as ices – frozen gases. As they approach the Sun and are heated, that material starts to boil off in streams of dust and gas, sometimes producing a spectacular appearance in the night sky.
Although it looks as if the tail is streaming out behind the comet as it moves, actually the tail always points away from the Sun, because it’s the Sun’s stream of particles (known as the solar wind) that drives the material away from the comet. As a result, when a comet is moving away from the Sun it actually travels tail-first.
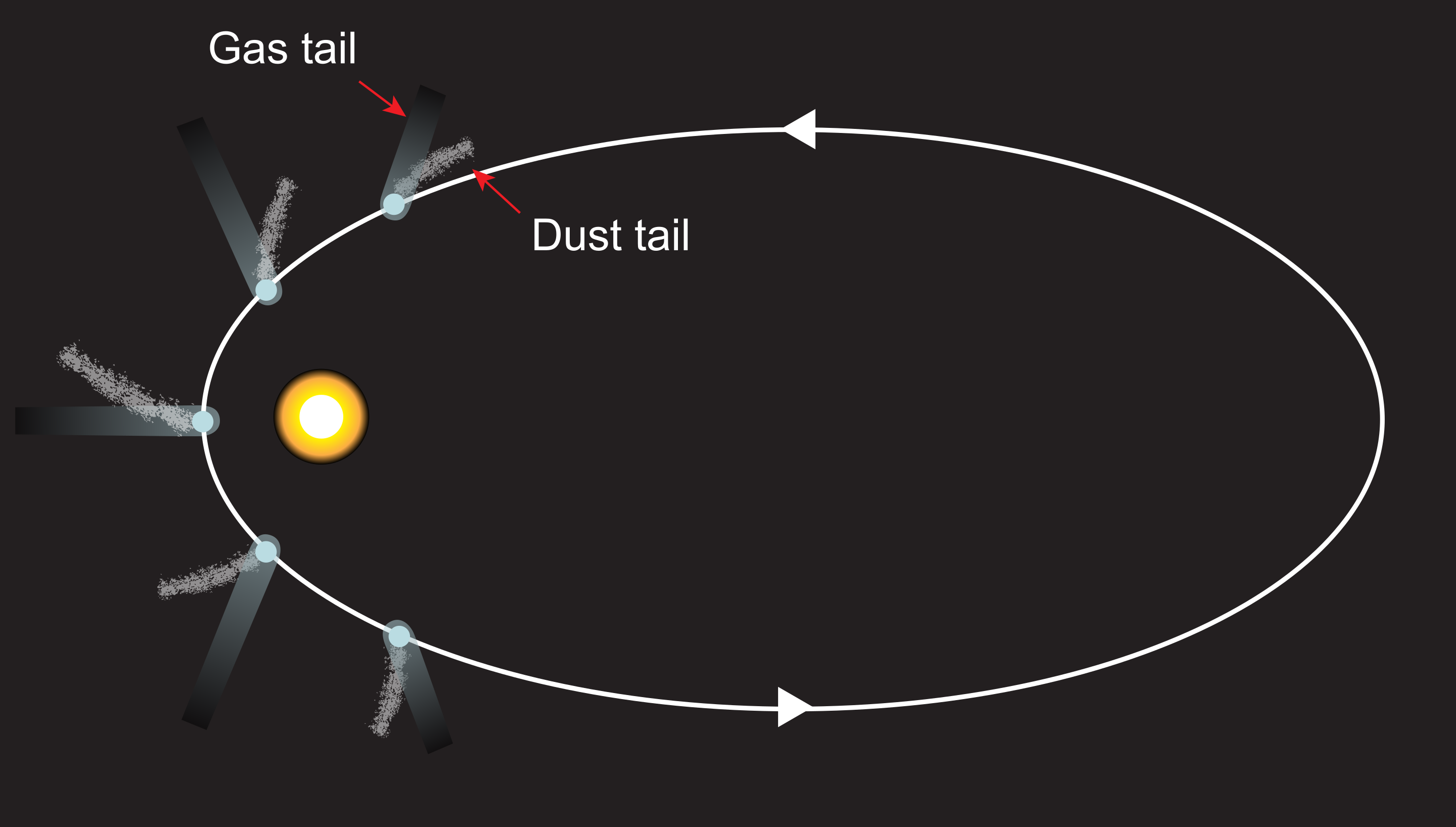
A big comet may be visible in the night sky for weeks or months before heading back toward the outer reaches of the Solar System. Once back out there, comets are invisible from Earth because they are very small and dark. However, it is believed that comets exist in large numbers in belts beyond the outer planets, known as the Kuiper Belt (30 to 50 astronomical units from the Sun) and the Oort Cloud (between 2000 and 50,000 astronomical units out).
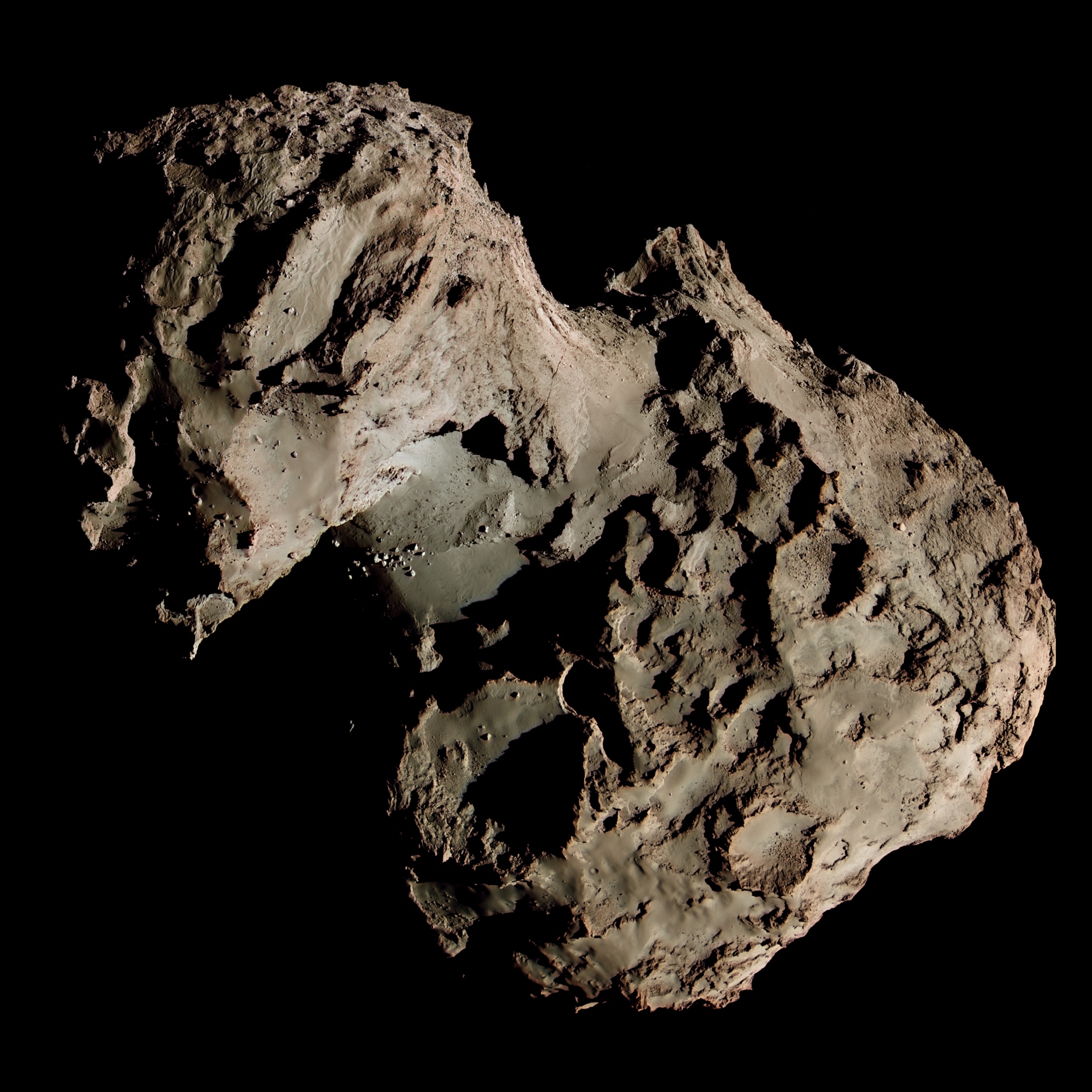
Humans are just beginning to be able to collect samples from comets – some spacecraft have brought back samples though few results have appeared at the time of writing. To preserve the volatiles, those samples have to be brought back in very tightly sealed containers. Analysing these precious samples requires rather special laboratory conditions. From what we know so far, their compositions may be quite similar to the compositions of the outer planets and to the Solar System as a whole.
Dwarf planets, asteroids, and meteoroids
The Solar System also contains a great range of more solid objects, without gaseous tails, that are smaller than planets.
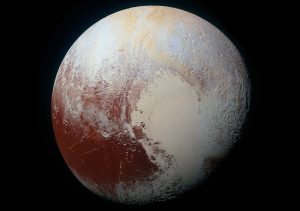
Objects with diameters from about 850 km up to about 2500 km are known as dwarf planets; these include Pluto, which was formerly classified as the outermost planet but was demoted from planet status a few years back. (A lot of Pluto enthusiasts protested.)
Asteroids are objects that range in size from 1 m to about 850 km in diameter. Many of these consist of materials that we might reasonably describe as rocks, but a proportion have a more metallic composition – they are mostly made of iron. They orbit the Sun in vast numbers, especially between 2 and 3.5 astronomical units from the Sun, between the orbits of the planets Mars and Jupiter, known as the Asteroid Belt. There is also a dwarf planet (Ceres) in the Asteroid Belt. There’s some suggestion that a full-sized planet might have formed in that part of the protoplanetary disk but the gravity of the largest planet Jupiter prevented planet formation. However, although over a million objects are known in the asteroid belt, their total mass is very much smaller than any planet. Only the largest asteroids are spherical; smaller objects are not spherical because their gravity is not strong enough to pull them into a rounded shape.
Chunks of solid material smaller than 1 m in diameter are known as meteoroids. Meteoroids are extremely numerous in the Solar System. Objects about a metre across hit the Earth quite frequently – perhaps about ten times per year. Smaller meteors are even more common. Meteoroids enter the Earth’s Atmosphere at high speed, where they typically burn up as a result of friction with the Atmosphere, producing “shooting stars” or meteors. (The word “meteor” originally meant anything that happened in the Atmosphere.) A meteoroid or asteroid that makes it through the Atmosphere to strike the Earth’s surface is called a meteorite.
Meteorites have been collected in large numbers and provide extremely valuable samples of the early Solar System. The reason they are so valuable is that the Earth’s surface layers are products of several billion years of dynamic activity, both within the Geosphere and in the Atmosphere and Hydrosphere, which have modified the Earth’s outer layers, the only parts we can get at. As a result, the oldest samples of the Earth’s crust give isotopic ages just over 4.0 Ga, whereas dated meteorites go right back to the origin of the Solar System at 4.6 Ga.
Meteorites have been classified into many different types, but the main categories are the following:
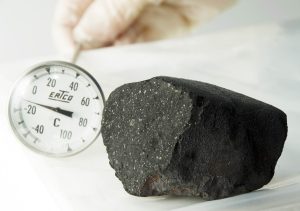
- The largest category (86%) of meteorites are chondrites, which appear to be collections of small particles fused together, including chondrules, small spheres that appear to have been drops of liquid that existed in the presolar nebula or protoplanetary disk. Chondrites are some of the closest materials we have to the original composition of the solid parts of the Solar System. Some even preserve volatile components. Their composition is often used to estimate the overall composition of the Earth, which is otherwise difficult to calculate because of our inability to sample the Earth’s deep interior.
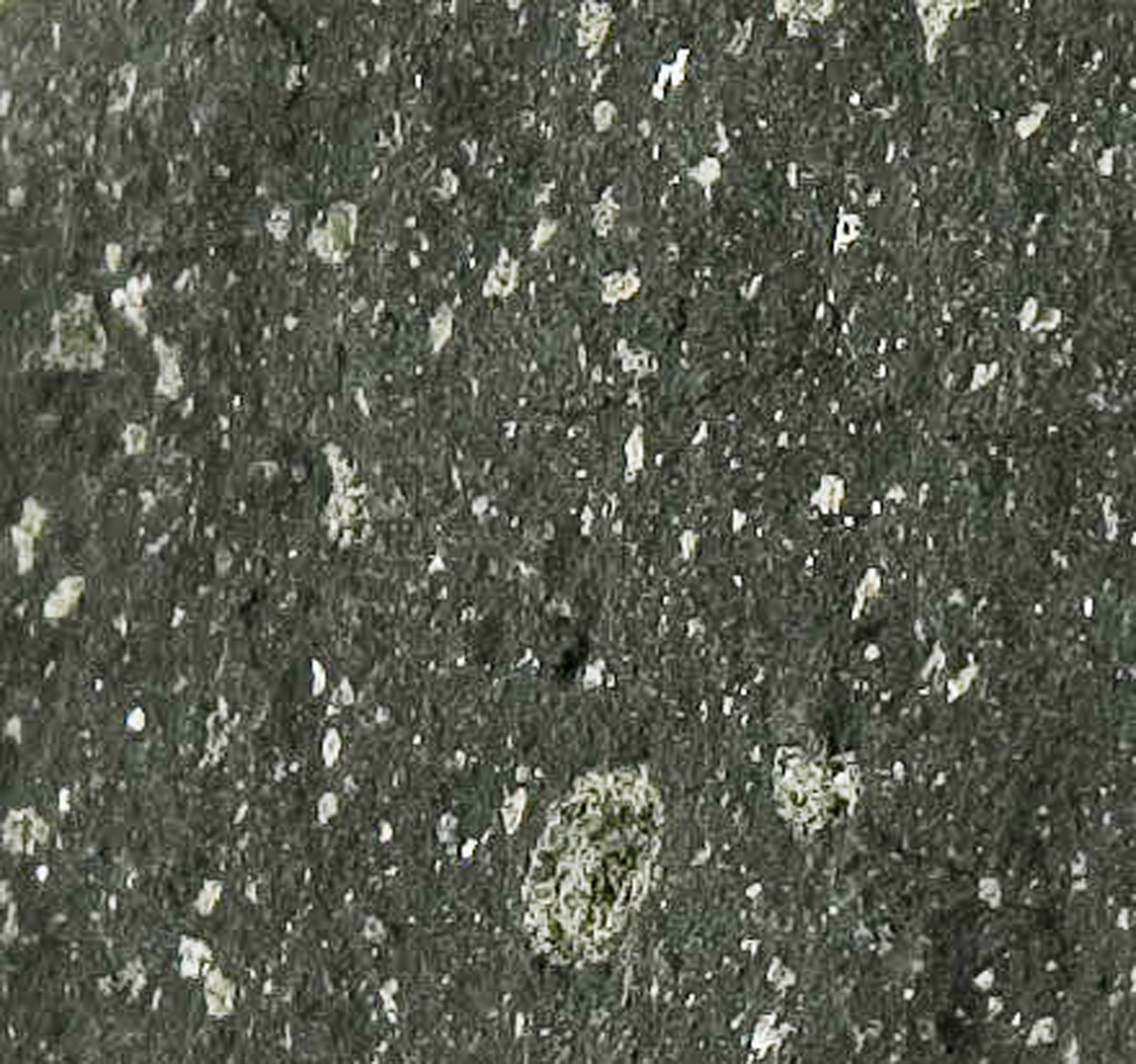
- Iron meteorites account for about 6% of the total. They are made of mixtures of the metals iron and nickel, and probably represent the cores of early asteroids, dwarf planets, or planets that disintegrated during early collisions in the Solar System. They give us additional insights into the makeup of Earth’s core, which is otherwise inaccessible.
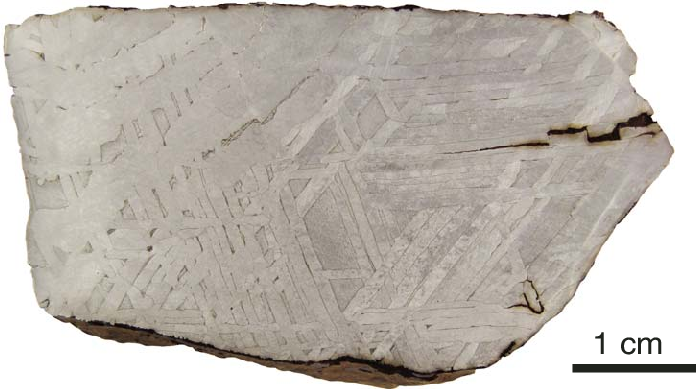
- Stony achondrite meteorites (~8% of the total) are composed largely of silicate minerals — combinations of silicon, oxygen, iron and magnesium mainly, and they correspond roughly in composition to what we know as the mantle of larger planets (see below). They probably represent remnants of the rocky mantles of planets, dwarf planets, or asteroids that broke up during intense collisions during the early history of the Solar System.
Planets
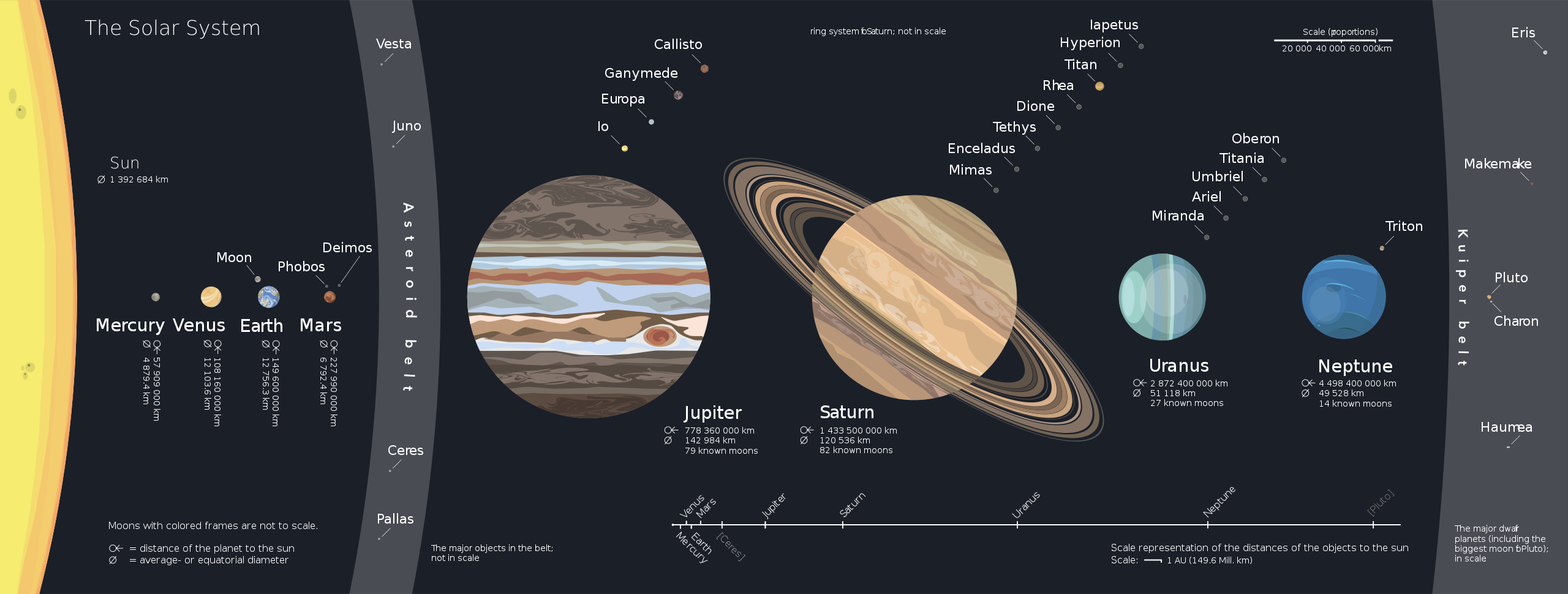
The best known and, apart from the Sun, largest objects in the Solar System are the planets, including Earth. There are eight planets in the Solar System, and as you can see from the diagram, they fall in two groups, separated by the Asteroid Belt. The outer four planets, known as jovian planets, are much larger than the inner four, known as terrestrial planets.
Jovian planets
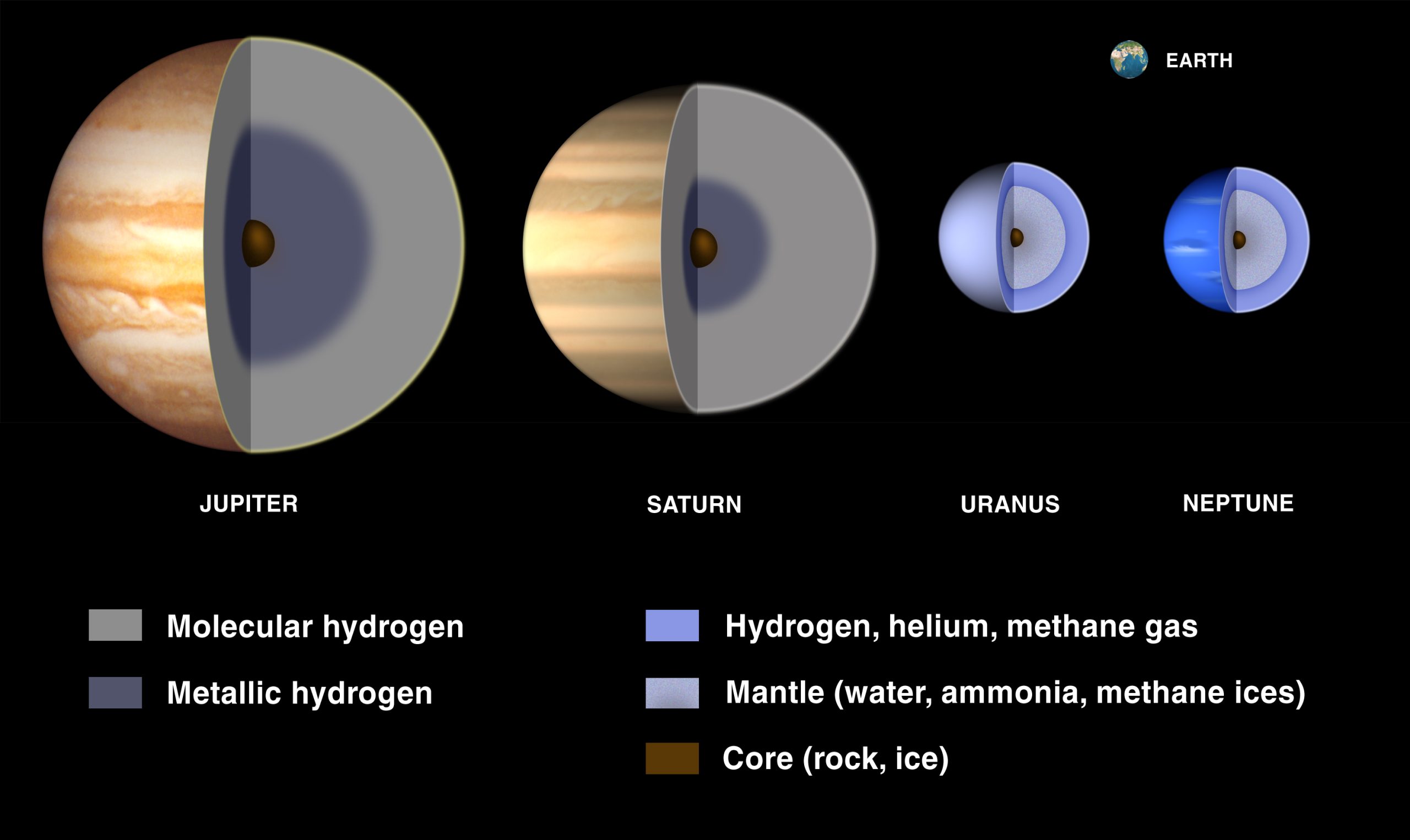
- Neptune
- Uranus
- Saturn
- Jupiter
The outer planets, named Jovian from the Latin name for Jupiter, are all much larger than Earth, and are believed to be made largely of substances that would be gases on Earth, the volatile components of the early Solar System. These include hydrogen and helium, the most abundant materials in the early Solar System, together with the hydrogen-rich gases methane and ammonia. Although these are all gases under Earth conditions, within the Jovian planets they mostly exist as liquids and solids because of the cold temperatures and high pressures that exist. In the largest of the Jovian planets, Jupiter itself, hydrogen probably undergoes a change at high pressure from a molecular liquid (in which hydrogen molecules, consisting of two hydrogen atoms H2, are present) to a form in which hydrogen behaves as a molten metal, in which there are no real molecules and the electrons are shared between all the atoms. Water may also be present in these planets, though likely as solid ice. The composition of the deep interiors of these planets is very speculative, but they may have central cores of solid material that is similar to the terrestrial planets.
Although broadly similar, the four Jovian planets have some differences. Neptune and Uranus are smaller and are thought to contain less hydrogen that Jupiter and Saturn, possibly because of their weaker gravity. The rotation axis of Uranus is “tipped on its side” relative to the Solar System and nearly all the other objects in it, suggesting that it was affected by a major impact in the early Solar System. Saturn is distinguished by a spectacular system of rings that are composed of huge numbers of small ice particles, and a smaller amount of rock. It has been speculated that the rings represent material from the protoplanetary disk that never condensed into natural satellites, or that they might be a natural satellite that broke apart under the influence of Saturn’s gravity.
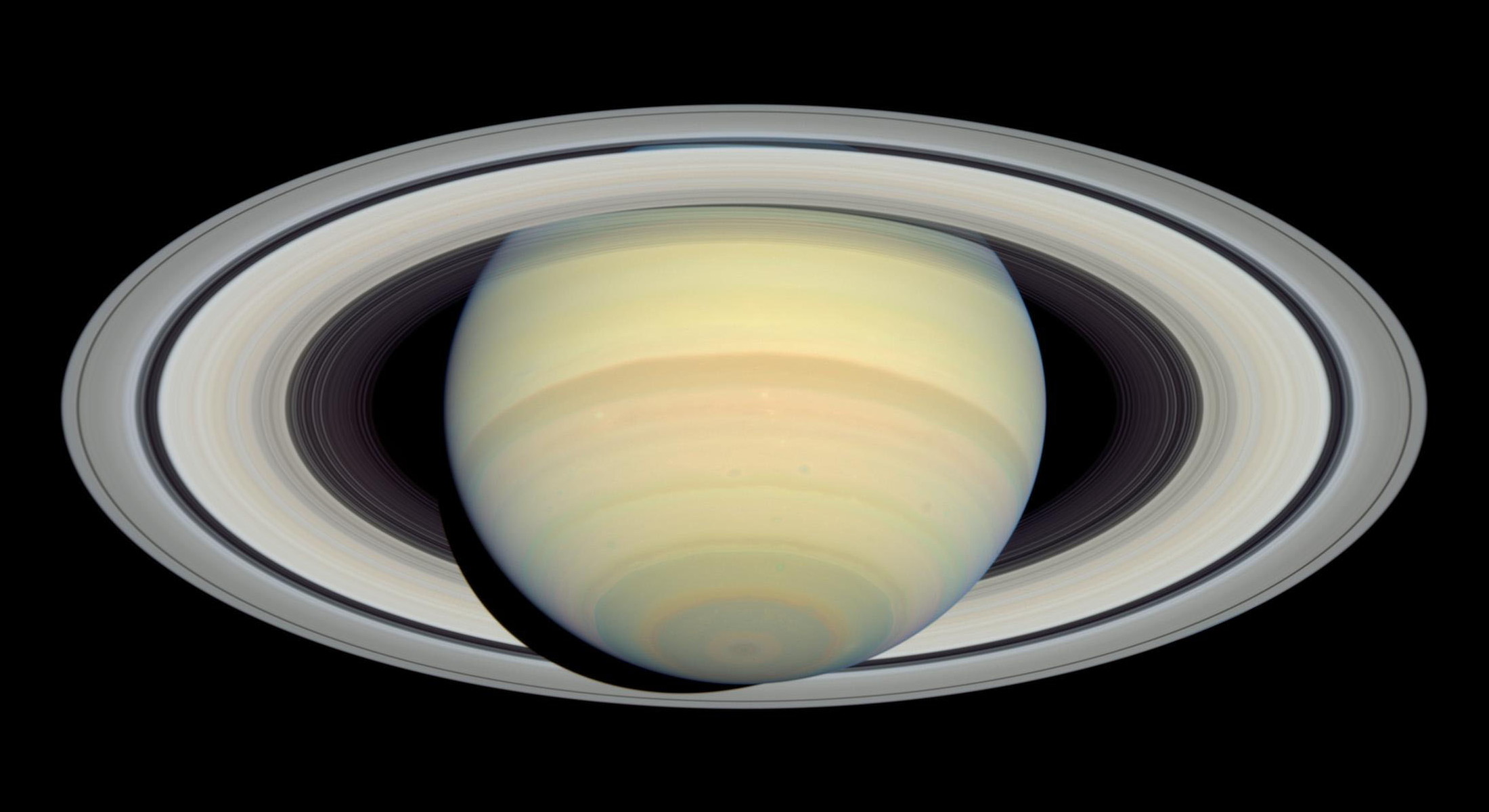
Terrestrial planets
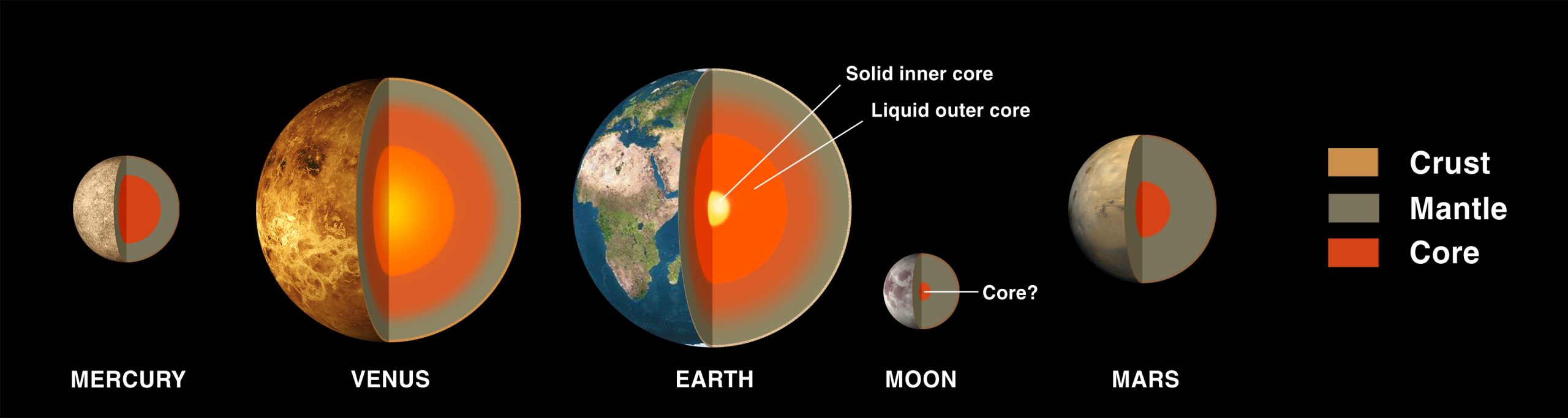
- Mars
- Earth (& Moon)
- Venus
- Mercury
The four inner, or terrestrial, planets are all much smaller than the Jovian planets, probably because they lost nearly all their volatile hydrogen and helium early in the Solar System’s history. The four inner planets are believed to share many features in common, and Earth’s natural satellite, the Moon, also shares some of those features. (The Moon is unusually large compared to other natural satellites in the Solar System, and may have had an origin more like that of the other terrestrial planets, forming with the Earth as a result of a violent collision in the early history of the Solar System.) Although we are unable to directly view the interiors of any of these planets, we have samples from the surfaces of the Moon and, of course the Earth, and we can also assess their internal structure by measuring the strength of their gravity and their magnetic fields. Achondritic and iron meteorites also give us clues as to what the interiors of terrestrial planets might look like.
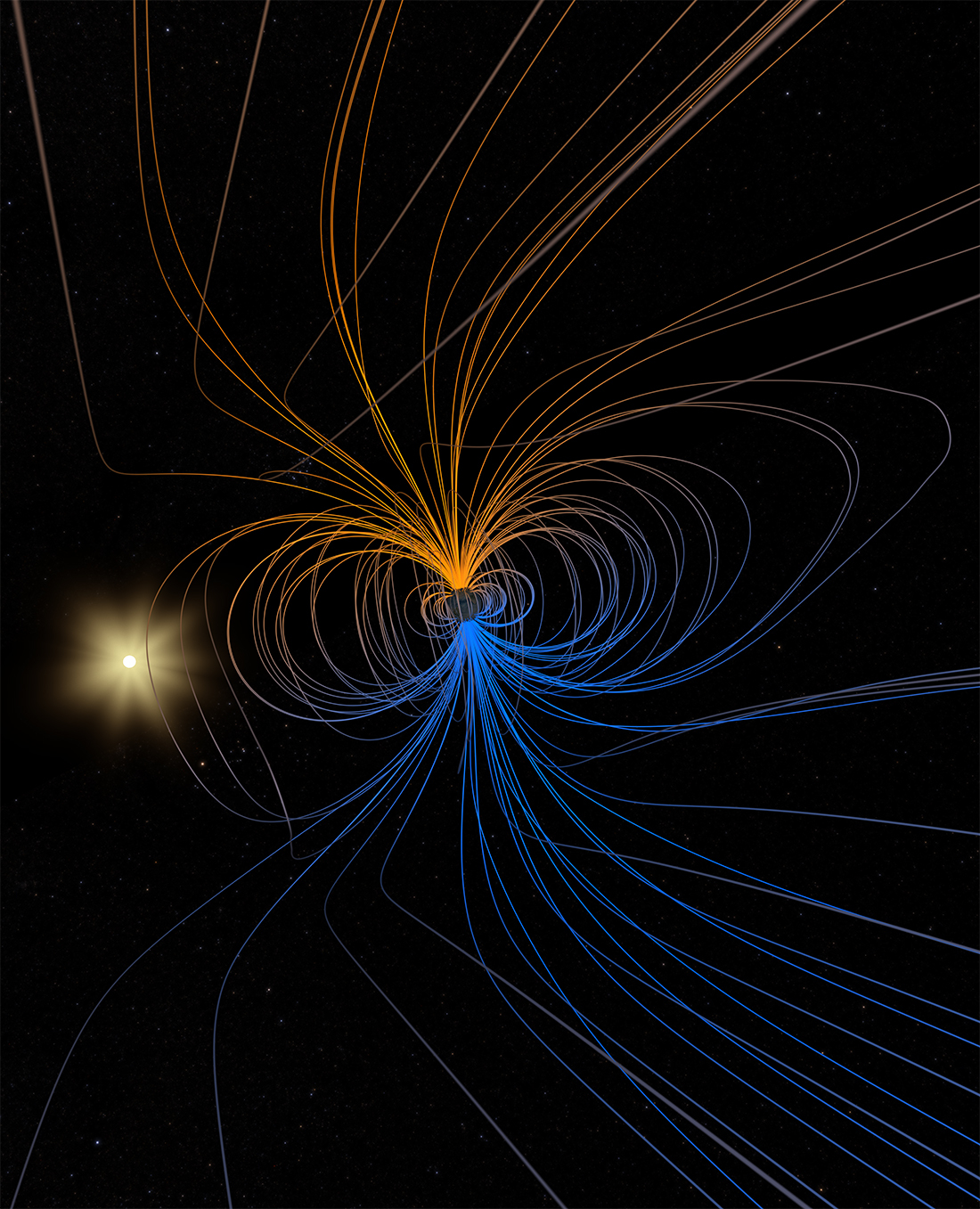
All these observations suggest that all five objects have a dense core composed mostly of metal: iron, mixed with a proportion of nickel. In the case of the Earth we know quite precisely that the diameter of the core is about half that of the whole planet, because seismic waves are routinely observed to bounce and refract off the core-mantle boundary. The Earth’s magnetic field tells us that the Earth’s core is mostly liquid, but there is probably a small solid inner core. Density measurements of Venus suggest that its core is closely similar to that of the Earth in size and composition. Mercury is much denser overall than Earth, so proportionally, its core is larger than that of Venus or Earth (though in absolute terms it is smaller). Mercury’s core is also thought to be part liquid and part solid. Mars and the Moon have relatively small cores, which are proportionally much smaller than Earth’s. Both are probably entirely solid, though intriguingly, we know from the magnetization of some ancient rocks in the crust of Mars (which can be measured by spacecraft) that the core of Mars was liquid at some point in its history.
Outside the core, each of these objects has a mantle, which is mainly solid, and which is dominated by four elements: oxygen, silicon, magnesium and iron, bound together in chemical substances called silicate minerals. Earth’s mantle is hot enough that it can flow slowly (by plastic flow) over time, allowing the surface part of the Earth to move around in a process called plate tectonics. As far as we know, none of the other terrestrial planets undergoes this degree of mobility.
The outer part of each planet, between 5 and 120 km thick, is called the crust. Initially, each planet’s crust was probably formed from magma extracted from the Mantle by the process of partial melting, and consisted of silicate minerals, though somewhat richer in silicon than the Mantle. However, each planet has a rock cycle that continuously modifies and resurfaces its crust, with the result that none of the original crust remains. Perhaps the most similar are Mercury and Earth’s Moon. Neither has a significant atmosphere, nor do they have significant mantle flow or melting at the present day. Their rock cycles are therefore dominated by meteorite impacts, and the crust of each has been affected by over 4 billion years of pulverization. As a result their surfaces are covered in craters. The Earth’s crust is partly covered by water, as was that of Mars in the past, and both planets have sedimentary rocks in their crusts that have been formed by the action of water in eroding material and transporting it over the surface. In addition, water has enabled life to develop on Earth, and additional large amounts of rock have been produced by the action of living things, both animals and plants. Plate tectonics has added to the complexity of the Earth, by stirring the Mantle and recycling the crust; portions of the Mantle continue to undergo partial melting, generating new magma and contributing to the variety of the crust. The planet Venus, though similar in size and overall composition to the Earth, is something of a mystery. Its surface is covered by dense clouds, which create a greenhouse effect so strong that the surface temperature is about 460°C, much too hot for water. Although spacecraft have been sent to Venus, our knowledge of its solid surface is very limited as a result of these challenges. We know little about its crust.
The atmospheres of the terrestrial planets are also variable. Mercury and the Moon are too small to retain a significant atmosphere. Mars and Venus have atmospheres of similar overall composition, overwhelmingly carbon dioxide (CO2): 95% in the case of Mars and 96.5% for Venus. In both cases there are a few percent of nitrogen (N2). Earth’s atmosphere is radically different: it consists of over 75% nitrogen, and 21% oxygen. Water vapour is third at 2–3% (though very variable) and argon, an inert gas, is the fourth most abundant component at about 1%. Carbon dioxide is fifth, at less than 0.03% historically, though this has been increased to 0.04% by human activity in the last 200 years. Carbon dioxide was undoubtedly much more abundant in the early Earth’s atmosphere, and oxygen was largely absent. The Biosphere has been responsible for these huge changes in the Earth’s atmosphere over geologic time, as we shall see in later sections.
Natural satellites or moons
Many of the planets have smaller bodies orbiting around them, known as natural satellites or “moons” [1]. New satellites are being discovered frequently by telescopes and spacecraft, so giving a precise number is difficult. At the time of writing there are 219 confirmed satellites, of which Saturn has the largest number (83). For a current list, visit:
https://en.wikipedia.org/wiki/List_of_natural_satellites
Almost all are small relative to their planet, although a few of the natural satellites that orbit the Jovian planets, together with Earth’s Moon, would be large enough to be considered planets in their own right if they were independently orbiting the Sun.
Most natural satellites orbit in the same direction, counterclockwise if viewed from above Earth’s North Pole, but a few of them have retrograde orbits, suggesting collisions or other close interactions in the early Solar System.
The Earth–Moon system
One natural satellite stands out as different from the others, and that is Earth’s Moon, which has a diameter over a quarter of that of the Earth. It would be regarded as a dwarf planet if it were independently orbiting the Sun. In some ways, the Earth–Moon system can be regarded as a double planet. This section will look at the Earth–Moon system in more detail.
Earth’s orbit and seasons
The most obvious features of the Earth’s motion, that dominate the lives of humans, are the spin of the Earth on its axis, which defines Earth’s day, and the travel of the Earth in its orbit around the Sun, which defines the year and the seasons. However, the Earth’s motion has several other parameters, which vary over timescales of thousands of years, known as Milankovitch cycles, after the Serbian scientist who hypothesized them. Milankovitch cycles will become important for us when we investigate the Earth’s weather and climate.
Eccentricity of Earth’s orbit
The Earth’s orbit is not a perfect circle; instead it is an ellipse, with the Sun at one focus. (Both foci lie on the long axis of the ellipse, but there’s nothing special at the other one.) The difference between the shape of the orbit and a perfect circle is measured by a quantity called the eccentricity. At present, the eccentricity of the orbit is about 1.7%, meaning that the Earth is about 3.4% farther from the Sun at aphelion (the farthest point) than at perihelion (the closest point). As the diagram shows, aphelion occurs in July and perihelion in January. (This may seem strange if you live in the northern hemisphere, where aphelion occurs in the warmest part of the year, but the seasons are mainly an effect of the tilt of the Earth’s axis, not the shape of its orbit.)
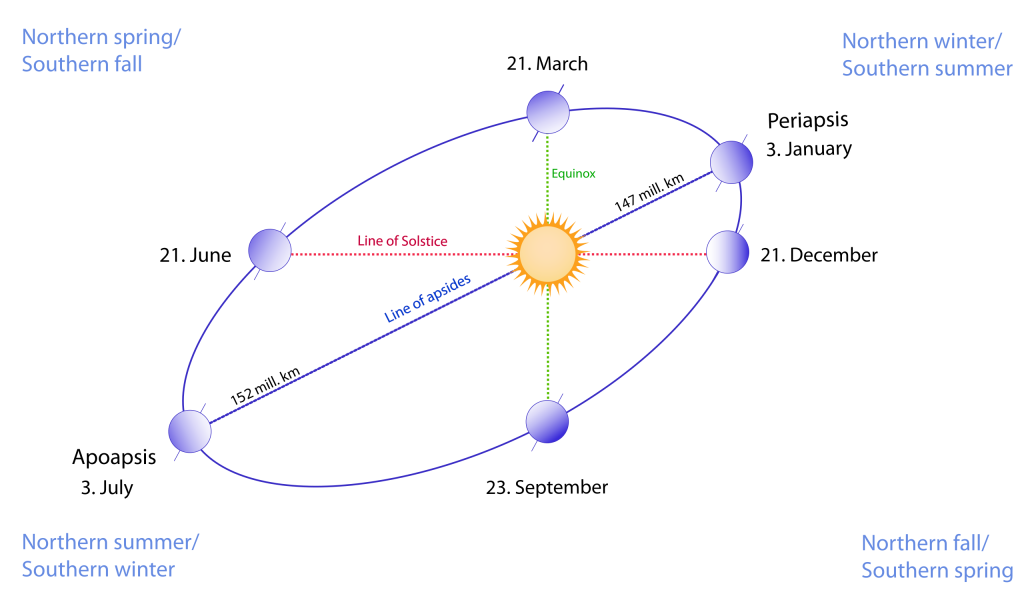
It turns out that the eccentricity is not constant. Because of the gravitational pull of Jupiter and Saturn, it varies between a low of about 0.3% and a high of about 6%. This variation occurs on a Milankovitch cycle of about 100,000 years; the eccentricity is currently declining.
Video Player
Movie illustrating variations in eccentricity. Credit: NASA/JPL-Caltech. Public domain. https://climate.nasa.gov/system/video_items/143_eccentricity_with_border.m4v
The changes in eccentricity cause very small changes in the total amount of energy received by the Earth. However, a much more significant effect is the distribution of energy over the year, and between the northern and southern hemispheres. At maximum eccentricity (6%), the Earth receives about 23% more energy at perihelion than at aphelion.
Tilt of Earth’s axis
The tilt of the Earth’s axis of rotation has a much larger effect on our weather and climate than the shape of the orbit. The axis is inclined to the plane of Earth’s orbit (the ecliptic) at an angle of about 23.4°, an angle known as the obliquity of the axis.
The obliquity is primarily responsible for Earth’s seasons. At the June solstice the North Pole is tilted 23.4° exactly towards the Sun. The northern hemisphere of the Earth therefore experiences summer, receiving a lot more energy than the southern hemisphere. An area around the North Pole, within the arctic circle, experiences constant daylight (the “midnight sun”) and an area around the south pole (within the antarctic circle) is in constant darkness. Conversely, at the December solstice, the South Pole tilts toward the Sun, and the southern hemisphere experiences summer while the north has winter.
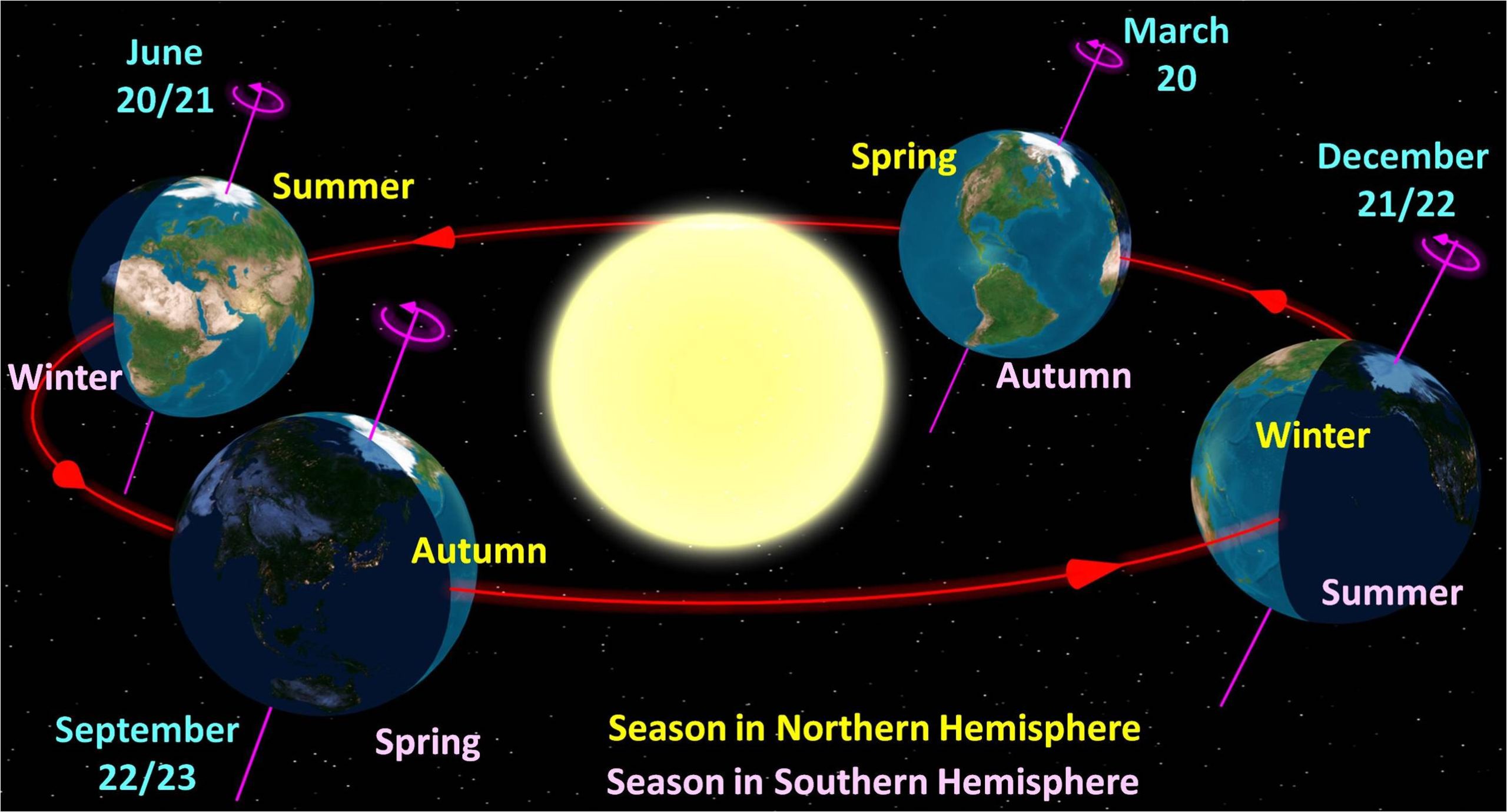
Between the two solstices are two equinoxes: moments when the tilt of the axis is “sideways-on” to the Sun. At the equinoxes all parts of the Earth have 12 hours of daylight and 12 hours of night.
The obliquity is not constant. It varies between about 22.1° and 24.5° in a cycle that lasts about 41,000 years. This Milankovitch cycle does not affect the total amount of energy received by the Earth, but it does affect the extremes of summer and winter climate. At times of high obliquity, summers are predicted to be a little hotter and winters are a little colder, if all other things are equal. Earth is currently midway between a maximum obliquity that occurred about 8,700 BCE; the next minimum is predicted to occur in about another 9,800 years.
Changes in obliquity (tilt) of Earth’s axis. Credit: NASA/JPL-Caltech. https://climate.nasa.gov/news/2948/milankovitch-orbital-cycles-and-their-role-in-earths-climate/ Public domain.
Precession
A third Milankovitch cycle involves the direction of the axis. The axis “wobbles” with an effect that has been compared to a wobbling spinning top, so that the axis points in different directions over time. This effect changes the timing of the equinoxes relative to the elliptical shape of the Earth’s orbit, and is known as the precession of the equinoxes.
Precession. Credit: NASA/JPL-Caltech. Public domain. https://climate.nasa.gov/climate_resources/251/axial-precession-wobble/
Like changes in obliquity, precession affects the distribution of energy between the northern and southern hemispheres. It’s probably mainly due to gravitational effects of the Moon and Sun on the Earth’s equatorial bulge. Strictly, the cycle of precession lasts about 26,000 years, but it interacts with some subtle changes in the shape of the Earth’s orbit as a whole, so the cycle of change in energy distribution is predicted to be about 23,000 years.
The shape of the Earth
The Earth’s shape is not a perfect sphere. Because of the rotation of the Earth, it is slightly flattened at the poles and bulges at the equator. There are smaller bulges and depressions at different places on the Earth’s surface.
The most common approximation for the shape of the Earth is an oblate spheroid, or ellipsoid of revolution, a slightly flattened sphere that looks circular when viewed from above the North or South Pole. Most map projections are based on an ellipsoidal model for the Earth. The most commonly used at the present day is WGS 84, which has an equatorial radius of 6378.137 km, and a polar radius about 21 km shorter, at 6356.752. This is a good approximation for the overall shape of the Earth.
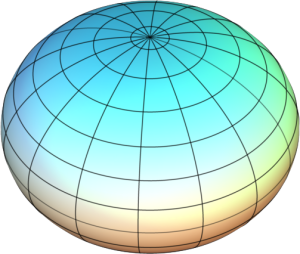
The Earth is not a perfect ellipsoid, however. It’s possible to define a closer approximation to Earth’s shape using very precise measurements of gravity, which pulls in slightly different directions depending on location. If we define a surface that is everywhere at 90° to the pull of gravity, that surface is known as the geoid. (In physics, the geoid is called an equipotential surface.) The geoid corresponds to the shape of the ocean surface if the Earth were entirely covered by water, and if there were no wind or tide to perturb the surface.
The shape of the geoid can be pictured using a map of the difference between the geoid and the ellipsoid, shown below.
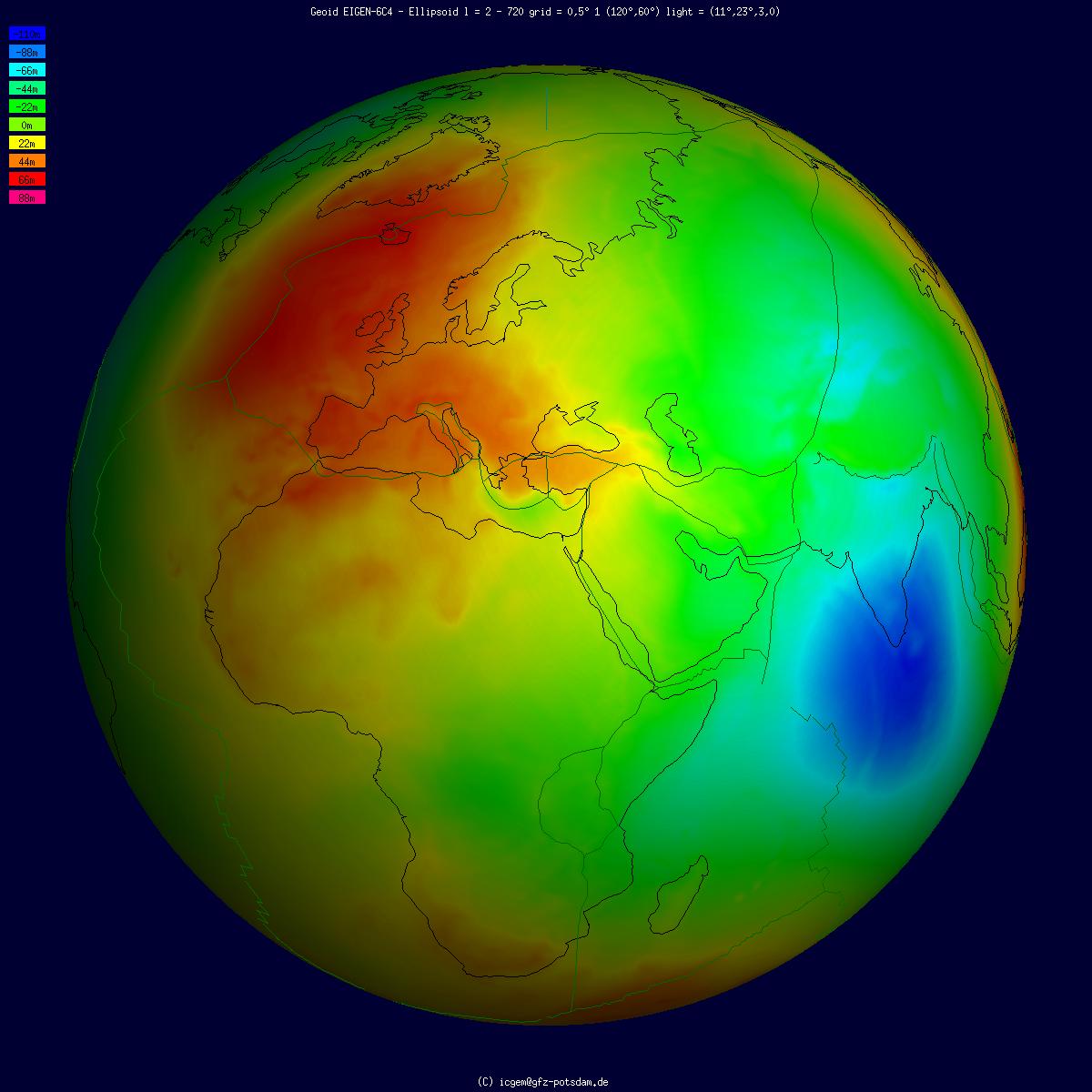
Moon’s orbit and tides
The diagrams above relate to the Earth’s average shape. However, the shape does not stay exactly constant with time, as it is affected by tides: changes in the shape of the geoid due to the gravity of the Moon, and to a lesser extent, the Sun. Tides distort the ellipsoidal shape of the Earth so that it is no longer circular when viewed from above the North or South Pole. The effect is much larger on water than it is on solid rock, so we perceive the tides as movements of water relative to the land, controlled by the monthly orbit of the Moon around the Earth and the yearly orbit of the Earth around the Sun..
The tidal effect of the Moon on the oceans is most easily understood by imagining an Earth covered in water to a uniform depth, with no continents to get in the way. Under these circumstances, the ocean water would be pulled into a prolate ellipsoid (roughly the shape of a Rugby or North American football) with one end pointing towards the Moon and one pointing away. The end pointing towards the Moon is easy to explain as the effect of the Moon’s gravity, but to understand why there’s a bulge on the opposite side we need to look at the whole Earth–Moon system in a little more detail.
Although we like to think of the Moon as simply orbiting around the Earth, in fact it’s more correct to say that the Earth–Moon system rotates around its combined centre of gravity or barycentre. Because the Moon is large, the barycentre is not at the centre of the Earth, but is displaced toward the Moon.
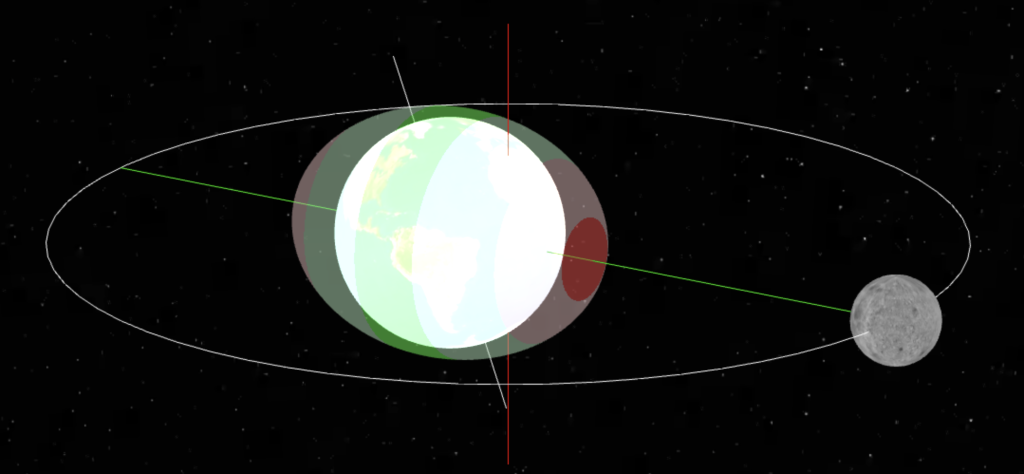
Both objects orbit around the barycentre. To explain the tidal bulges non-mathematically, recall that the equilibrium orbital speed of an object is defined by Kepler’s third law, which shows that the rate of rotation should decrease as distance from the barycentre increases. The ocean water on the side of the Earth closest to the Moon is orbiting a little more slowly than would be predicted, and it tends to ‘fall’ toward the Moon. Conversely, the water on the far side of the Earth from the Moon is travelling faster than the equilibrium rate for that distance; inertia tends to make it travel in a slightly straighter path, making it look as if it’s pulled away from the Earth. (More mathematically, this effect is expressed by analysing the differences between the Earth’s and the Moon’s attraction vectors at different locations in the Earth–Moon system.)
On this idealized Earth, the surface of the ocean would rise and fall about 54 cm during the day, producing a tidal range of just over a metre, because the Earth is spinning inside the ellipsoidal mass of water.
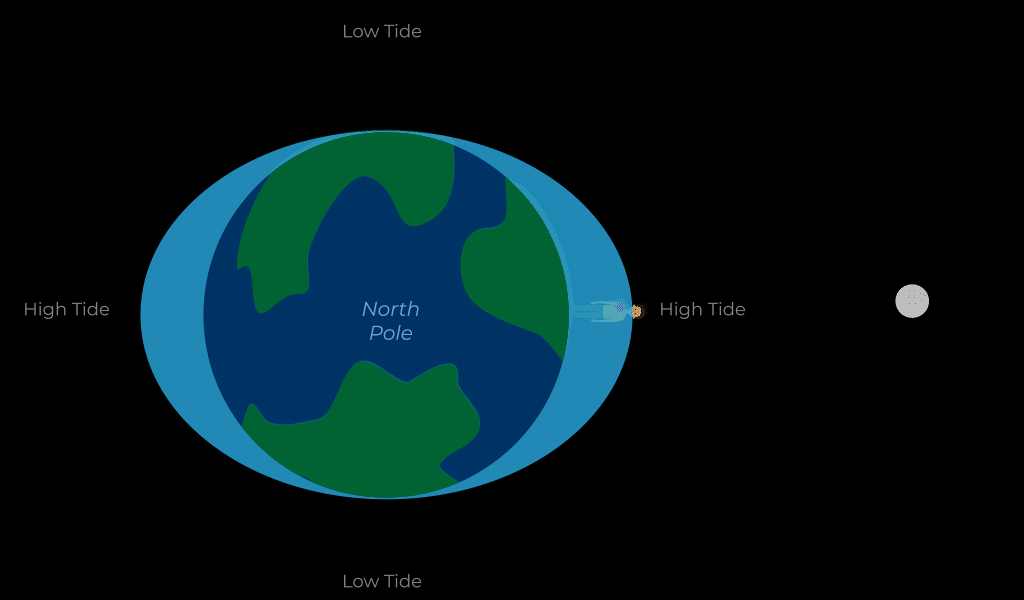
The time between high tides is a little over 12 hours, because in the time it takes the Earth to turn from one tidal bulge to the next, the Moon has moved along in its orbit.
The Sun also has a tidal effect, just under half of that of the Moon (because, though more massive, it is much further away).
- When the Earth, Sun, and Moon all lie in a straight line, the two effects reinforce each other, so the theoretical rise and fall is 79 cm. High tides are much higher and low tides are lower. This phenomenon is called a spring tide, not because it occurs in spring, but because the water “springs up” fast. Spring tides actually occur about every two weeks, around the time of full moon and new moon.
- During the intervening times, when the Sun and the Moon are 90° apart in the sky, their effects work against each other, so the high tides are less high, and low tides are less low. This phenomenon is a neap tide.
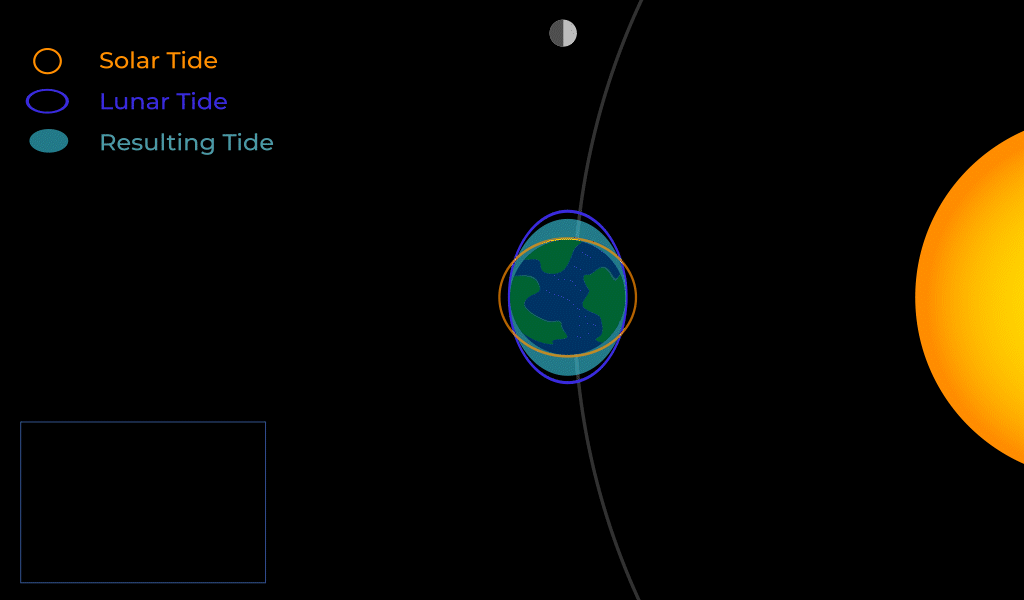
In the real Earth, the presence of continents and changes in the depth of the ocean have large effects on the tidal cycle, amplifying it in some areas, reducing it in others, and delaying the spring and neap tides by a couple of days relative to the positions of the Moon and Sun. Tides are revisited in the section on the hydrosphere, where some of these more complicated tidal effects are described.
The Sun
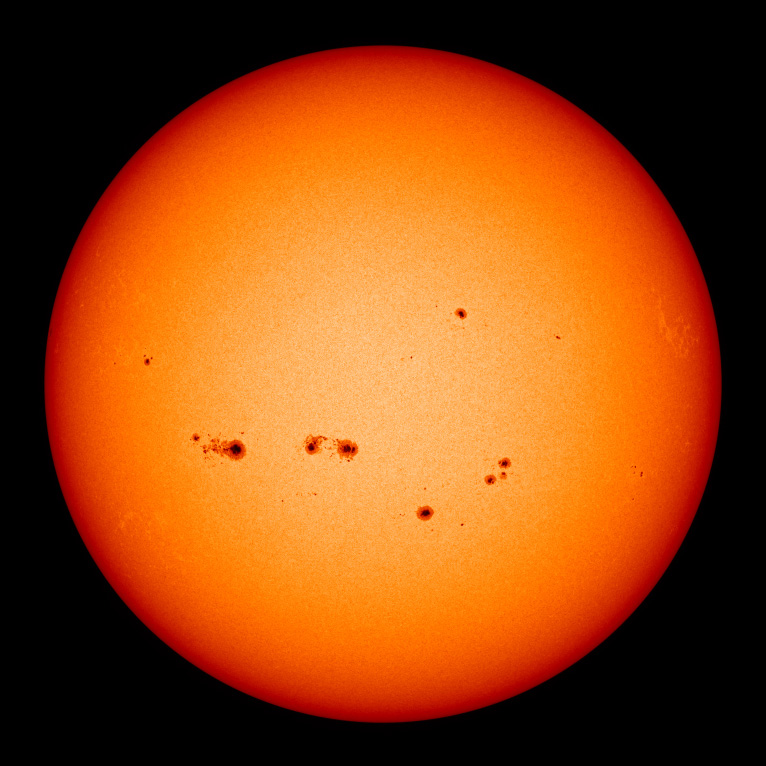
From the point of view of the Earth, the Sun is the most important other object in the Solar System. In this section we will look at the Sun’s energy output, and then consider how that energy arrives at the Earth’s surface.
Solar output
The Sun is a pretty average star. Its radius is about 700,000 km, 109 times that of the Earth. Its mass is about 300,000 times that of the Earth, or 1030 kg. The Sun is mostly made of hydrogen (~75%) and helium ~(24%). Within the Sun, these elements exist in the form of plasma, a form of matter in which all the electrons are separated from the atomic nuclei because of the high temperature. The energy source for the Sun is nuclear fusion (the same process that occurs in a hydrogen bomb) which converts 4 hydrogen atoms to one helium atom, releasing large amounts of energy in the form of electromagnetic radiation. Most of this energy is produced in the innermost part of the Sun, termed the core, which extends to about one quarter of the radius of the Sun as a whole. Energy is conducted through the Sun and eventually radiated from the photosphere (the visible surface of the Sun) as electromagnetic radiation. Its total energy output is about 3.8 x 1026 W (watts; one watt is rate of energy flow equal to one joule per second). The Sun also radiates matter, particles that escape from the surface to form the solar wind, flowing outwards through the Solar System and carrying a relatively small amount of additional energy.
Electromagnetic radiation behaves as waves under some circumstances, and as particles called photons in others. The electromagnetic radiation from the Sun includes:
- Radio waves
- Microwaves
- Infrared radiation
- Visible light
- Ultraviolet radiation
- X-rays
- Gamma rays
All these types of radiation travel at the same speed through space (the speed of light, c = 3 x 108 m/s), but they are distinguished by their different wavelengths (λ) and frequencies (f). These quantities are related by the equation
c=fλ
…so that as the frequency goes up the wavelength decreases.
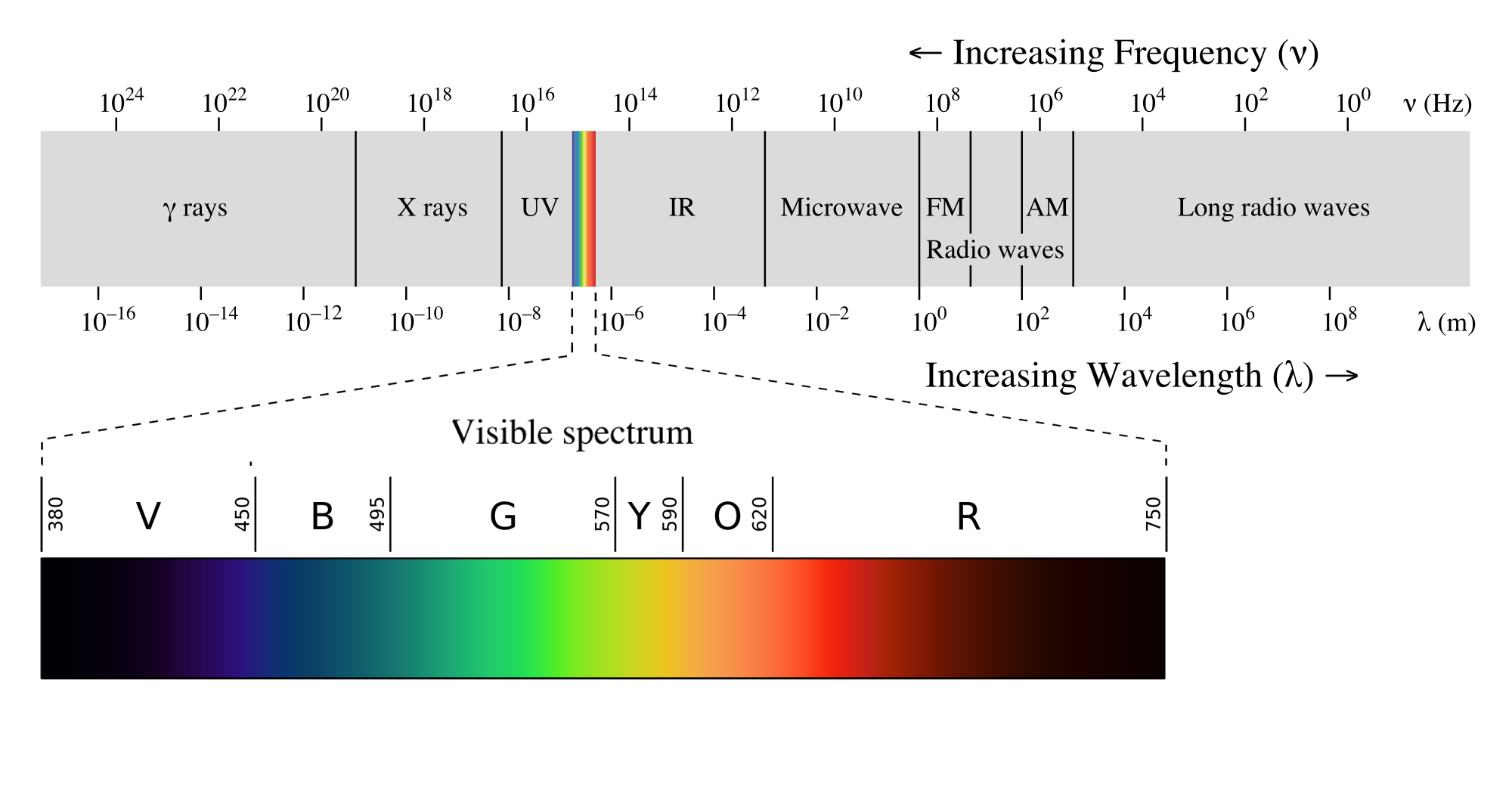
The solar output also varies over time because of changes within the Sun itself that seem to be related to variations in the number of sunspots observed on the photosphere. Sunspots are dark patches probably formed by turbulence within the Sun. These variations occur in an 11-year cycle known as the sunspot cycle. The actual variation is tiny (less than one part in a thousand) – much smaller than variations associated with the Earth’s orbit.
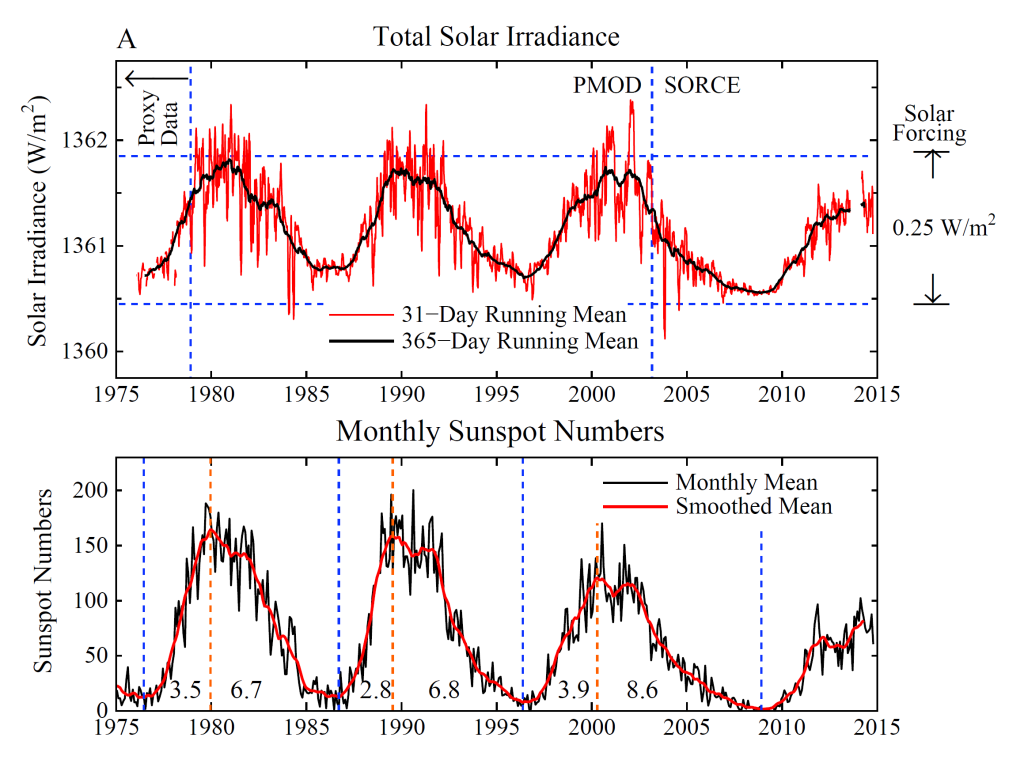
Some slightly longer-term effects are also known. Systematic sunspot records go back to around 1600 CE, and a period from ~1650 to ~1715, known as the Maunder minimum, was characterized by very low sunspot numbers. We also know that at that time the solar wind was less intense; this has been determined from the amount of radioactive carbon in the atmosphere, preserved in samples of wood from that time. From this it is known that the Maunder minimum also coincided with a decline in the strength of the solar wind and it has been suggested that this may have corresponded to a decrease in irradiance by as much as a quarter of one percent. Similar measurements of carbon isotopes have been used to make estimates of sunspot numbers and corresponding small irradiance fluctuations going back about 2000 years.
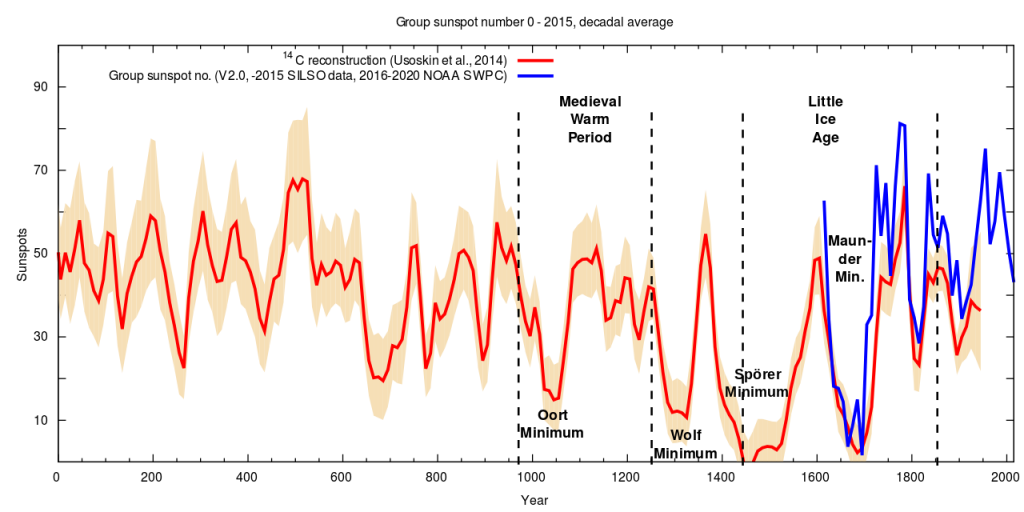
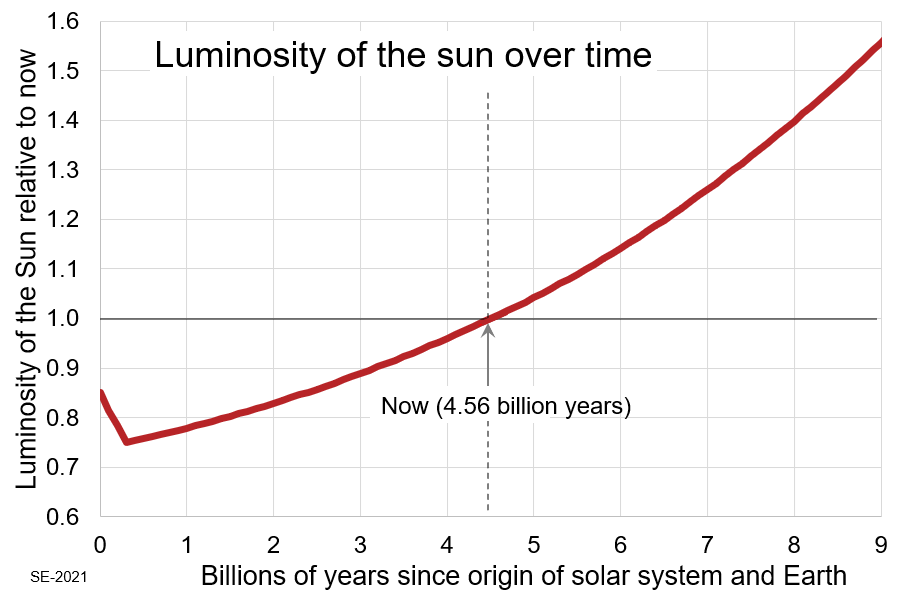
Much slower changes have probably affected the Sun’s output over the lifetime of the Solar System. Modelling of the nuclear fusion processes that fuel the Sun, combined with astronomical observations of similar stars at various stages in their life history, suggest that the Sun’s output has steadily increased since the origin of the Solar System, and will continue to increase for billions of years into the future. However, the rate of increase is far too slow to be detected on a human time-scale.
Sun’s energy reaching the Earth’s surface
As the Sun’s energy spreads through space it of course becomes diluted. By the time it reaches the Earth, the concentration of power (known as the solar energy flux or irradiance) is about 1365 W/m2 – watts per square metre; this means that each square metre of Earth’s surface could receive 1365 joules of energy per second. The actual number varies somewhat depending on the distance of the Earth from the Sun in its elliptical orbit.
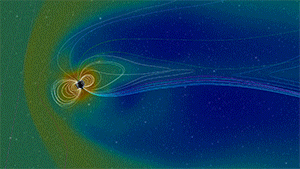
The stream of particles emitted by the Sun (the solar wind) is mostly obstructed by the Earth’s magnetic field, but this represents only a small part of the Sun’s energy output.
The energy reaching the Earth consists of a variety of different frequencies and wavelengths (as shown by the yellow curve in the diagram below). Much of the energy is in the visible and infrared wavelengths, but there are also ultraviolet and radio waves. Some wavelengths are filtered out by the Earth’s atmosphere. The red curve shows the typical amounts of energy at different wavelengths that reach the Earth’s surface. Note that a lot of ultraviolet radiation is filtered out by ozone (O3), and that other distinct wavelengths are removed by water vapour (H2O), oxygen (O2), and carbon dioxide (CO2).
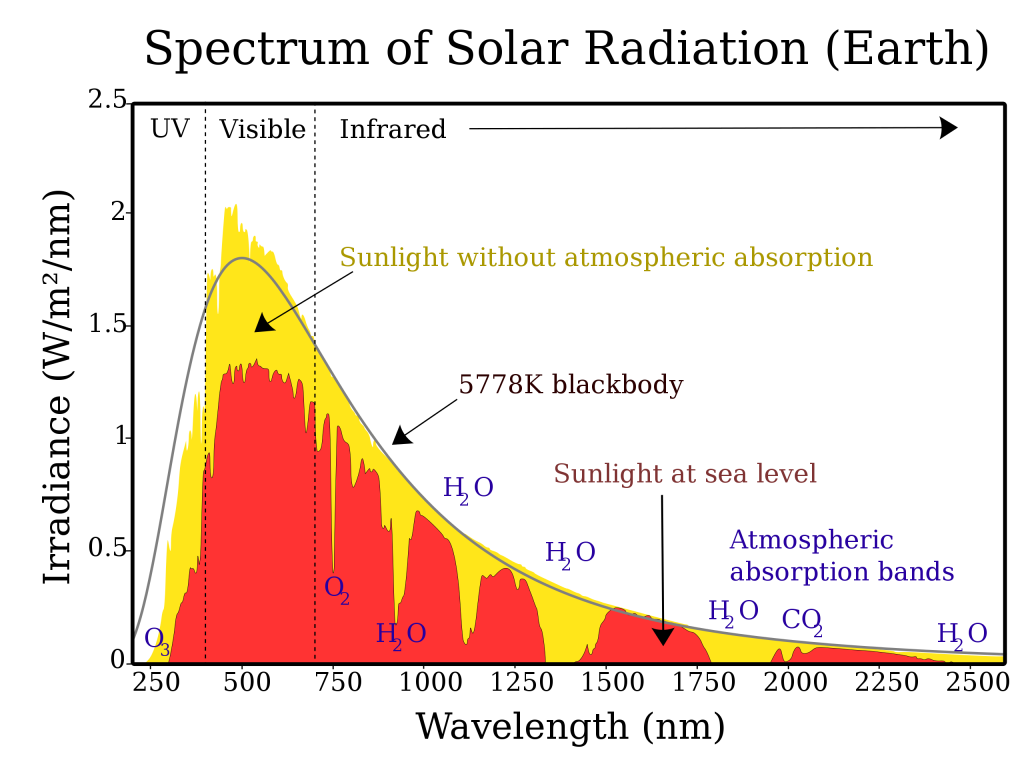
As a result of all this absorption, the final energy flux at the Earth’s surface at sea level when the Sun is overhead is about 1000 W/m2. However, the Sun is only ever overhead at any one point on the Earth’s surface. At all other locations on the sunlit side of the Earth, the energy flux is reduced because the Sun’s rays come in at an angle, and are therefore spread out over larger areas. This diluted energy flux is known as insolation and of course varies with latitude and with the seasons.
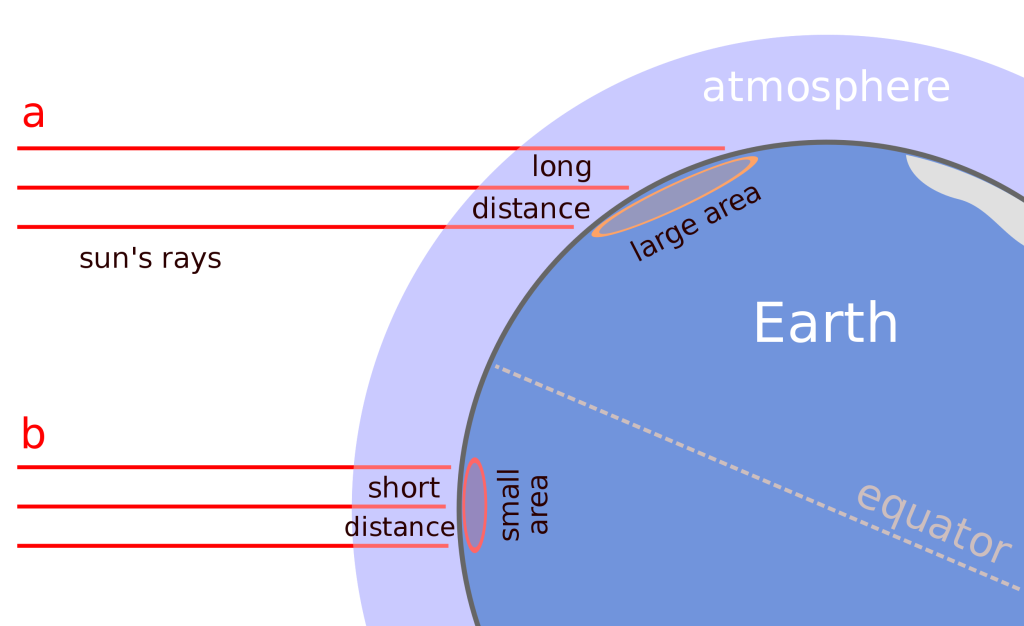
The Energy cycle on Earth
What happens to that ~174,000 TW of power (that’s 174,000 TJ/s, or 1.74 x 1017 J of energy per second)? We need to look at Earth’s energy cycle.
Available energy
In order to understand the energy cycle, we must look at all the energy available to the Earth system. In addition to solar energy, there are two other significant energy sources to consider. One we have met already: the tides. The Moon, and to a lesser extent the Sun, move water around in the oceans, providing a source of energy from gravitational forces. The total amount of energy is estimated at about 3 TW, much smaller than the radiative energy from the Sun.
Another source of energy at the surface of the Earth is geothermal energy: heat from within the Earth. This comes from two sources: breakdown of radioactive substances inside the Earth, and leftover energy from the formation of the Earth during the origin of the Solar System. The total rate of energy flow is about 47 TW. Volcanoes and hot springs are the most spectacular manifestation of geothermal energy on land, but they account for only about 0.3 TW. Submarine volcanoes release more energy: up to 11 TW by some estimates. The remaining geothermal energy (at least 36 TW) exits the Earth by conductionspread variably over the Earth’s surface, averaging about 70 mW/m2 (milliwatts per square metre).
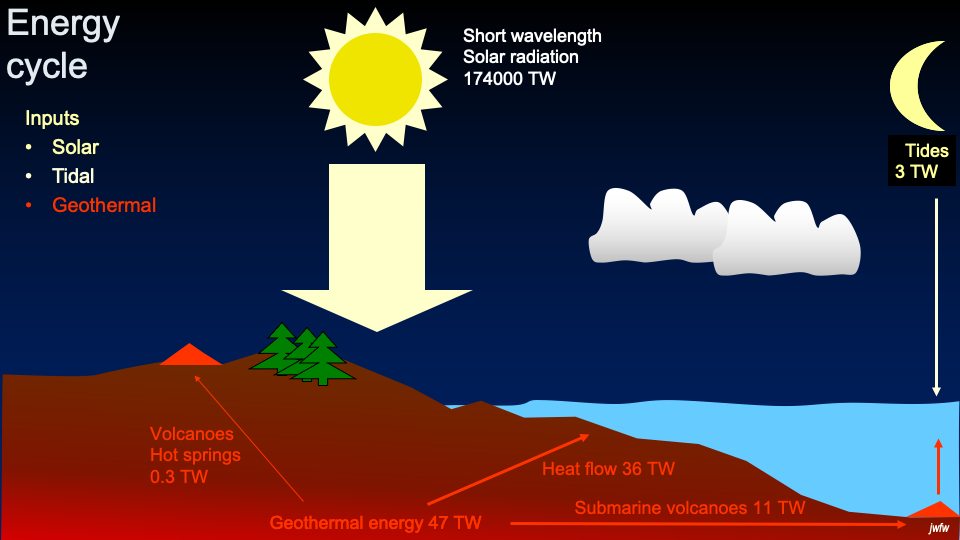
From these numbers, it is clear that by far the largest input to the energy cycle is energy radiated from the Sun. In the next paragraphs we will look at where this energy goes.
Energy reflected back into space
About 30% of the incoming energy, or 51,000 TW, is simply reflected back into space. The value 30% is known as Earth’s albedo, which is about average for a planet. For comparison, the albedo of Venus, which is covered by white clouds, is about 75%. The albedos of the Moon and Mercury, both bare rock with no atmosphere, are both 12%.
Earth’s albedo varies a little through time, because ice and clouds are much more reflective than water or forests or deserts. As the Earth warms, the proportion of the surface covered by glacier ice decreases. This in turn decreases the albedo, leading to further warming. This kind of self-accelerating process is known as a positive feedback loop.
Energy re-radiated as infra-red radiation
Much of the rest of the Sun’s radiation, about 46% or 81,000 TW is absorbed and converted to heat energy by the Atmosphere, the Hydrosphere, and the Geosphere. It warms these materials, and they re-radiate the energy, but as lower frequency, longer-wavelength infra-red radiation, also known as radiant heat. This radiation eventually makes its way back into space, but some of it is intercepted on the way by the Atmosphere. Certain atmospheric gases, particularly carbon dioxide and methane, can absorb some frequencies of infra-red radiation, leading to the temporary storage of heat, warming the Atmosphere. This effect is similar to the behaviour of the glass in a greenhouse, which is transparent to visible light, but which blocks infra-red radiation, warming the interior. It’s therefore known as the greenhouse effect, and gases that absorb infra-red are known as greenhouse gases. When the proportion of greenhouse gases in the Atmosphere goes up (as has been happening for the last 2 centuries as a result of human activity), the increased trapping of heat leads to warming of the Atmosphere.
Melting and evaporation of water
Most of the remaining solar energy, about 40,000 TW or 23% of the total, goes into phase changes in the hydrosphere. Ice, liquid water, and water vapour are known as phases of water. Converting ice to water, and water to steam, absorbs a lot of energy (known as latent energy). Both these processes occur in the hydrologic cycle, with the result that energy is temporarily stored in liquid water and water vapour, another energy storage bank. Eventually, as we have seen in our look at the hydrologic cycle, the water in the Atmosphere falls as precipitation – rain or snow – and the stored energy is released, contributing to the overall flow of infra-red radiation out of the Earth system into space.
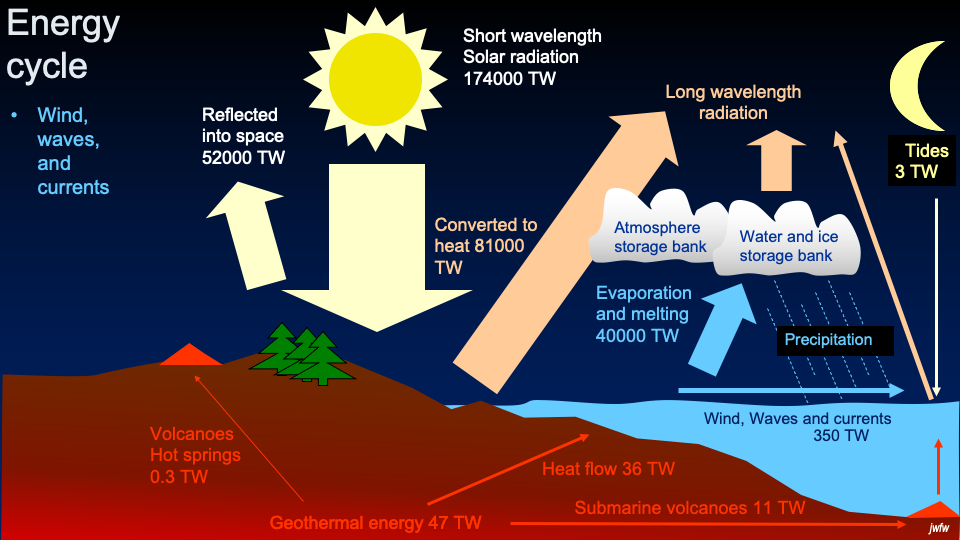
Energy flow in the Atmosphere and oceans
A proportion of the incoming solar energy, by heating the Atmosphere and oceans, causes convection currents in both. In this process, heat energy is converted to kinetic or mechanical energy. This is the fundamental mechanism of origin of wind in the Atmosphere, and of ocean currents in the Hydrosphere. As we shall see when we look in more detail at the Atmosphere and the oceans, the rotation of the Earth also has a major role in modifying the patterns of circulation in both. These movements involve about 350 TW of power. Tidal energy gets mixed in to the kinetic energy of the ocean, too.
Eventually, all this energy is converted back into heat through processes that include friction against the solid surface of the geosphere, and viscous behaviour of the air and water.
Energy captured by living things.
A proportion of solar energy, about 40 TW, is captured by the Biosphere. This primarily occurs in the bodies of plants through photosynthesis, a process in which captured solar energy is used to split carbon dioxide from the Atmosphere and store energy in carbon compounds. These carbon compounds make up both the bodies of organisms and their energy source. The energy is released to organisms in the processes of respiration, in which carbon is recombined with oxygen and returned to the Atmosphere as carbon dioxide. These processes are covered in more detail in a later chapter on carbon and energy in the Biosphere.
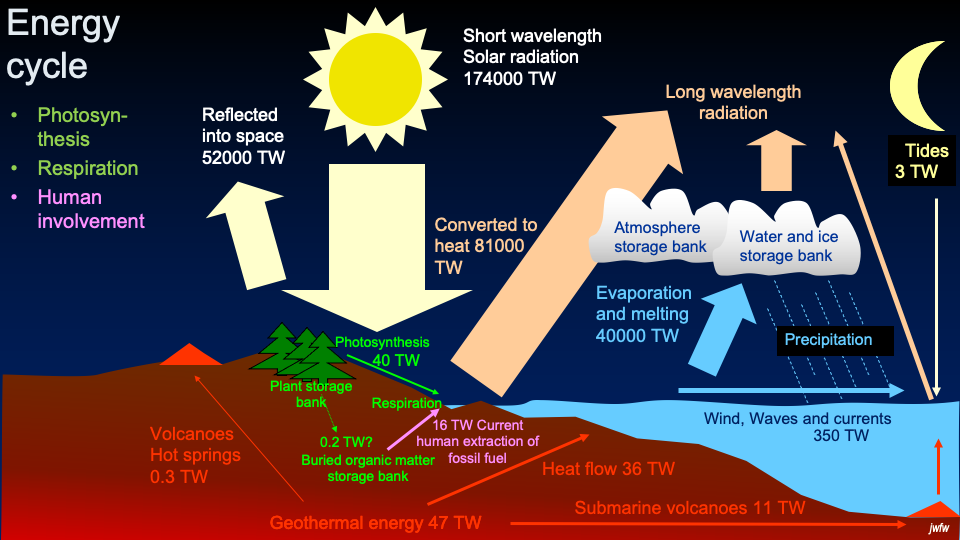
A small proportion of the carbon captured by photosynthesis ends up being buried in the Geosphere, mostly in sediments, which may become lithified into sedimentary rocks. The exact rate of burial is difficult to quantify, and has varied through geologic time, but is estimated at only 0.2 TW, less than 1% of all the carbon extracted by photosynthesis.
Humans use this store of energy when they exploit fossil fuels — coal, oil, and natural gas — burning them in oxygen to release the stored energy and return carbon dioxide to the Atmosphere. Humans are currently burning fossil fuels at a rate of about 16 TW, perhaps 80 times faster than the rate at which carbon is being stored in the Geosphere. For this reason, fossil fuels are known as non-renewable resources. The accelerated return of carbon dioxide to the Atmosphere has consequences for the Earth’s climate, consequences that humans are only starting to grapple with.
- We write moons with a lowercase m to distinguish it from the Moon which is Earth’s one natural satellite ↵
The Sun and all of the natural objects (including planets, their natural satellites, dwarf planets, comets, asteroids and meteoroids) in its orbit.
A large, approximately spherical body (diameter greater than 850 km) of rock, liquid and/or gas in orbit around the Sun or another stars
A body in the Solar System with diameter larger than 850 km yet smaller than the planet Mercury
A small object which orbits the Sun in the Solar System, ranging in size from 1 m to 850 km
Bodies in the Solar System smaller in size than asteroids, with diameters less than a metre.
A small body in the Solar System with composition including large components of ices; comets develop a tail as they approach the Sun along eccentric elliptical orbits
Objects in the solar system that orbit around a planet
A system that exchanges neither matter nor energy with its surroundings
Interstellar clouds of dust and gas
The hypothesized nebula where the Sun and Solar System condensed from more dispersed dust and gas
An abbreviation for Giga-annum, meaning 1,000,000,000 (one billion) years ago
Isotopes of an element are atoms that have the same atomic number but different atomic mass, because of different numbers of neutrons in the nucleus
The solid Earth
All of the material in the primeval Solar System which did not condense directly into the Sun. This material would instead form the planets, their natural satellites, the asteroids, meteoroids and comets.
Natural satellites of planets are sometimes known as moons.
Element 1, the most abundant element in the Universe and in the Sun
A process in which the two light atomic nuclei join together to form a single more massive nucleus, releasing energy
Element 2, an inert gas
The four outermost planets in the Solar System, having small rocky cores wrapped in thick atmospheres and a multitude of natural satellites.
The four innermost planets of the solar system believed to have iron-rich cores surrounded by rocky mantles, without the thick atmospheres characteristic of the outer planets.
A theory that the Earth has a lithosphere that is divided into relatively rigid moving plates that interact along plate boundaries
The breakdown of rock material at the Geosphere surface due to the action of the Atmospher, Biosphere, and or Hydrosphere.
The removal of material from a site of weathering on the Earth's surface.
The focus points of an ellipse are two special points that lie on the long axis of the ellipse, symmetrically on either side of the centre at a distance apart f = √(p2-q2) where p and q are the major and minor diameters of the ellipse. The more eccentric the ellipse, the farther apart are the two foci.
A scientific description or hypothesis drawn directly from observation or experiment
The resistance of an object to changes in movement, requiring that a force is necessary to change its velocity
The average distance between the Earth and Sun; 1.5 x 109 metres
The length of the long axis in relation to the length of the short axis of an ellipse; a measure of the difference between the ellipse and a perfect circle.
Matter (plasma specifically) released from the surface of the Sun that carries small amounts of energy throughout the solar system.
A belt of mainly comets hypothesized to orbit around the Sun beyond the orbit of Neptune
A spherical collection of comets outside the orbits of all other planets, at distances between 2,000 tand100,000 astronomical units from the Sun.
A dwarf planet once considered the ninth planet from the Sun.
A belt of small objects (asteroids) whose orbit lies between those of Mars and Jupiter
Meteoroids as they enter Earth’s atmosphere. Friction with the atmosphere causes a glowing tail to form in the wake of the meteoroid.
The extraterrestrial rock mass that has reached the Earth’s surface through impact.
A type of meteorite characterized by small, roughly spherical particles of glass or former glass, known as chondrules
Small spheres interpreted as the remains of liquid droplets from the early Solar System.
A minority of meteorites, composed primarily of iron material probably derived from the cores of early proto-planetary bodies in the Solar System.
The central part of the Earth below about 2900 km from the surface, composed largely of metallic iron
A silicate-bearing meteorite that lacks chondrules (spherical glassy fragments); achondrites
probably represent the remains of mantle material from planets in the early Solar System.
The layer within the Earth or other inner planet that overlies the core and makes up the majority of the planet, composed chiefly of magnesium- and iron-rich silicates
The third planet from the Sun in the Solar System, and the principal object of study for geoscientists; the only planet known to possess life.
Relating to planet Jupiter and the other outer planets.
The fifth planet from to the Sun, and the largest
The eighth planet from the Sun.
The seventh planet from the Sun. Uranus is a Jovian planet with a rotational axis with obliquity of nearly 90°
The sixth planet from the Sun; a Jovian planet noted for its visible ring system
Associated with the Earth
Earth's only natural satellite; also (with lowercase m) any natural satellite of a planet
A major discontinuity and seismic reflector ~2900 km below the Earth's surface, separating the silicate mantle from the metallic core
The third planet from the Sun known for its similar size to Earth; the brightest planet in Earth's night sky
The nearest planet to the Sun, and the smallest planet.
The fourth planet from the the Sun
Naturally occurring crystalline solids whose composition includes groups of silicon and oxygen atoms, typically in a covalently bonded tetrahedral arrangement
Continuous deformation of crystalline materials that takes place after a yield stress is exceeded
A thin, surface layer of the Earth that lies above the Moho; differentiated into thicker, lighter continental crust and thinner, denser oceanic crust
Melting of rock material to produce a mixture of solid and liquid.
The process by which igneous, sedimentary, and metamorphic rocks arise through cycling of material through the Earth's outer layers
The absorbtion of outgoing infra-red radiation by atmospheric gases, leading to heading of the Earth’s atmosphere.
A gas in which each molecule combines one atom of carbon with two of oxygen; formula: CO2
Element 7. In the molecular form N2, the most abundant gas in Earth’s atmosphere.
Element 8. In the molecular form O2, the second most abundant gas in Earth’s atmosphere.
Water in its gaseous state, a colourless invisible gas
An inert gas, element 18
The Earth system that comprises all living things and their non-living remains
The clockwise rotation (when viewed from above Earth's north pole) that a minority of objects in the Solar System show.
Repetitive variations in the Earth’s tilt, axis orientation and elliptical orbit shape, that cause climate forcing.
The point in the Earth's elliptical orbit that is closest Sun
The furthest from the Sun of the Earth in its elliptical orbit.
The plane of Earth’s orbit around the Sun.
The angle at which the Earth’s axis of rotation is tilted towards the ecliptic.
Two times in an Earth year at which one of the poles is directly tilted towards the Sun.
The northernmost area of the Earth which experiences constant daylight at the summer solstice, and constant darkness at the winter solstice
The southernmost area of the Earth that experiences constant darkness at the Summer solstice, and constant daylight at the Winter solstice
A time in an Earth year when which the tilt of the Earth's axis is perpendicular to the line between the Earth and the Sun, resulting in equal lengths of daylight and darkness
The rotation of the Earth’s tilted axis of rotation, which causes a change in the position and timing timing of the solstices and equinoxes relative to the axes of the Earth's elliptical orbit
A ellipsoid with circular symmetry about an axis which is shorter than its other radii; a flattened sphere.
A three-dimensional shape with only elliptical cross-sections
A equipotential surface that approximates the Earth's surface. Gravity pulls at 90 degrees to the geoid surface everywhere.
The change in Earth’s surface elevation (particularly water surfaces) due to changes in the gravitational pull of the Moon and Sun.
The centre of gravity of a system; the pivot point about which a system rotates when freely spinning.
The vertical distance between high and low tide levels at a point on the Earth's surface.
A tidal phenomenon caused by the alignment of the Moon, Sun, and Earth along a single line, producing a maximum tidal range and accentuating tidal effects.
A tidal phenomenon caused by a 90 degree angle between the Sun and the Moon, resulting in reduced tidal range.
A high-temperature form of matter wherein the electrons of each atom are freed from the nuclei.
The visible surface of the Sun.
A common type of energy movement characterized by oscillating electric and magnetic fields, including radio, infra-red, visible, and ultraviolet light, x-rays and gamma rays
The distance between the two successive maximum values or crests in any wave-like phenomenon.
For a wave-like phenomenon, the number of waves that pass a point in one second; measured in hertz (Hz).
A dimmer patch on the photosphere of the Sun associated with magnetic storms
The 11 year-long rise and fall in sunspot activity across the surface of the Sun, associated with a minor change in irradiance
An interval during the late 17th to early 18th century CE when sunspots were at a minimum
The rate of energy input from the Sun that could theoretically be received by a unit area of the Earth’s surface.
The solar energy flux, typically measured as a rate of energy flow per square metre at the top of the Earth's atmosphere
The amount of solar radiation received by a unit surface area in a given time period
Energy originating from within the Earth, either from radioactive decay or from the orignal heat trapped at the planet's formation
A process of movement of heat or electricity which does not involve the movement of the material through which it travels
The proportion of light that is reflected from the surface of a substance
A cycle in which the products of a process act as inputs increasing the activity of the same process
Heat energy in the form of electromagnetic waves with relatively wavelengths from ~1 mm to ~700 nm, the limit of visible light
Infra-red radiation released by heated objects
A compounds in the Earth’s atmosphere which absorbs infra-red radiation, preventing or delaying its escape into space.
The transition of a compound from one phase to another, typically in response to a change in temperature or pressure.
The part of a system that is separated from other parts by distinct boundary surfaces; for example water exists in three phases: solid ice, liquid, and vapour, which do not mix.
Energy released or absorbed in phase changes.
A cyclic motion of gas or liquid as it is heated. As the material is heated, it rises due to the lower density. Once it moves far enough from the heat source, it begins to cool, causing it to sink again, returning it to the heat source.
Having to do with movement
related to movement and forces
The resistance between two solids moving against one another
Describes liquids with high ability to resist flow
The production of reduced carbon compounds from water and carbon dioxide using light as an energy source, carried out by cyanobacteria, many algae, and plants.
The oxidation of reduced (organic) carbon compounds by an organism to yield energy, carbon dioxide and water
Sedimentary material that has solidified into sedimentary rock
Relating to sediment, material derived ultimately from weathering, that is transported and/or deposited as grains on the surface of the Geosphere
Carbon-rich substances found in the geosphere and formed from the remains of past-living organisms. Fossil fuels contain sufficient concentration of reduced carbon to produce usable heat when burnt in air.
Materials such as fossil fuels and minerals which do not form at a rate fast enough to replace their consumption by humans.