Chapter 4: Skeletal Muscle Structure, Form for Function
Brian R MacIntosh and R. John Holash
Brian R MacIntosh, R John Holash
Faculty of Kinesiology, University of Calgary
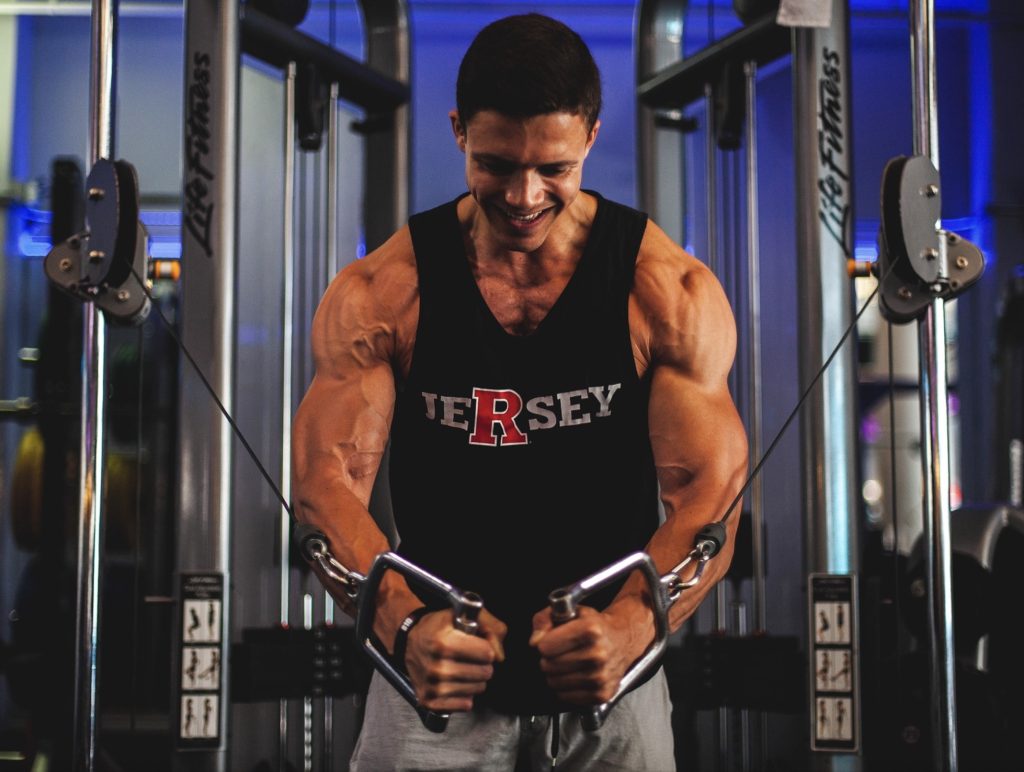
Learning Objectives
After studying this chapter, you will be able to:
- Describe the general structure of skeletal muscle
- Describe the structure and function of connective tissue
- Describe the structure of motor units and relate to the central nervous system
- Describe the tendon and aponeurosis structure and function
- Identify structures associated with the neuromuscular junction
- Illustrate a single muscle fibre, myofibril and sarcomere at the microscopic level
- Describe the role played by membrane proteins
- Name and identify the function of a number of proteins that are important to muscle
Key Terms
tendon, aponeurosis, inscription, fascicle, myocyte, motor unit, connective tissue, sarcomere, cytoskeleton, myofilament, myofibril, sarcoplasmic reticulum, channels, ion pumps, transverse tubules, neuromuscular junction, end-plate, triad, terminal cisternae
Introduction
Skeletal muscle is an excitable contractile tissue that makes up 20-35% of total body mass in women and 30-45% in men (Janssen et al. 2000). As such it is an incredibly important tissue of the body. Many features of life can be directly attributed to skeletal muscle: body shape, speech, synchronized and coordinated movement, locomotion, posture, breathing, controlling the direction the eyes are looking and manual manipulation of objects. With such a broad range of functional roles, skeletal muscle must have an adaptable structure that in its elementary state can respond to neural activation with that may achieve a variety of outcomes. These outcomes include: maintain force or limb position, allow shortening or lengthening for joint motion, generate power and achieve or dissipate work. In this chapter we will look at muscle structure from a macroscopic perspective, considering how the cells are aligned and connected to bones through tendons. We will progressively zoom in on the tissues as we look at the subcellular structures and the specific proteins that make up muscle. Before we get to specific structural analysis, consider the Case Study presented next.
Case Study: Consider the Holiday Turkey
Ever since taking anatomy in a Kinesiology class, Rachel could not eat meat without relating it to muscles. She was enjoying turkey dinner with her family and picking delicately at the drumstick in front of her. There was a layer of oily under the skin. She noticed how the individual muscles pulled away from each other, maintaining a distinct shape. The Achilles tendon was connected to the large muscles on one side and ended bluntly where it had been cut away from the calcaneus. She knew that muscle fibres, too small to see separately were bundled by connective tissue into fascicles. She also recognized that dark meat, like that on the thigh and drumstick was composed of muscle fibres with high oxidative capacity, whereas white breast meat was composed of fast-twitch fibres with a high glycolytic capacity. With careful observation, she could see nerves and blood vessels disappearing into the meat near the end of the bone.
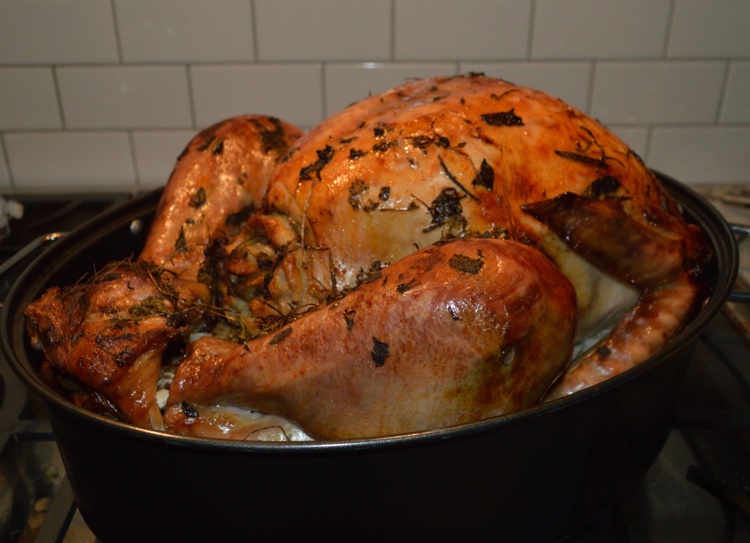
Gross Structure of Skeletal Muscle
All muscles are composed of individual muscle cells, also known as myocytes or muscle fibres, which are long, tubular cells, surrounded by a basement membrane and a thin layer of connective tissue called endomysium. At each end, the muscle cell is typically connected to tendon, which in turn connects to other tendons and bone. Contraction of the muscle fibre applies a force to the bone, which generates a moment about the joint. This moment (moment is a rotational force whose magnitude is the applied force times the perpendicular distance to the axis of rotation) can result in motion if the force is sufficient to overcome the load. Skeletal muscles are voluntary muscles in that we can decide when to cause contraction, controlling the timing and magnitude of in a coordinated fashion using the motor nerve pathways (see Chapter 3 on Neural Control). Skeletal muscle cells are striated; they have a banded appearance under the microscope, similar to cardiac muscle. This banded appearance arises from the regular pattern of sarcomeres along each cell. Sarcomeres are described later in this chapter.
In some cases, the whole muscle is much longer than any single fibre within the muscle; for example, sartorius and semitendinosus muscles, both of which extend from the pelvis to the upper parts of the shin. In these cases, individual fibres insert either into a connective tissue band called an inscription or the fibres are of varied length and occupy different portions of the full length of the muscle, interdigitating with each other. In the case of inscriptions, all fibres of a given segment are of a similar length and there may be two or more groups of fibres separated by inscriptions. It is generally thought that no muscle fibre is longer than about 12 cm in the average sized human body (MacIntosh et al. 2006). In contrast, some of the muscles of the body may be 30-40 cm in length. In many muscles of the body, individual fibres may only be a few cm in length.
Tendons connect our muscles to bone so that when a muscle contracts, the bone is pulled via the tendon. In some cases, the muscle fibres are oriented in line with the tendon, and in some cases, the angle of orientation of the muscle fibres is different from the line of direction for the tendon. In the latter case, the muscle is considered to be pinnate (see muscle shapes below).
Muscle Fascicles, Tendon and Aponeurosis
The gross structure of skeletal muscle is essentially what you see when the skin is removed, exposing muscle. The skeletal muscles obviously have a variety of shapes: long and thin (neck strap muscles) or long and wide (hamstrings); some muscles lie flat against the underlying body structures (abdominals) and some bulge, showing their large size (gluteals). Regardless of their shape, the muscles of the body serve specific functions related to generation of force, and movement. Function may be as diverse as to maintain posture, walk, run, throw, manipulate or support. Specialized functions exist for the muscles around the eyes and within the ears. Muscles give our faces expression, and in some cases, can cause wrinkling of the skin. This wrinkling can be overcome with injection of botulinum toxin (botox), an inhibitor of neuromuscular transmission. This practise is not recommended because there are long-term negative consequences of this treatment (Fortuna et al. 2011; Yaraskavitch et al. 2008).
A Simple Model of Muscle Contraction
Simple models of muscle have been presented in the research literature. Most of these originate in the models proposed by A.V. Hill (Hill 1953), before the sliding filament theory of muscle contraction was proposed (see Chapter 5). Figure 4-1 presents a common model of muscle contraction with a contractile element, in-series elastic component and a parallel elastic component. This simple model proposed by Hill has anatomical and physiological correlates that help us understand the relationship between muscle structure and function. Essentially, the contractile element represents the muscle fibres, or more specifically the myofibrils and the contractile proteins. The series elastic component represents the tendon, which lies in series between the muscle fibres and the bone. The parallel elastic component represents a series of tissues that support the muscle in the passive state: membrane, connective tissue and titin. These structures will be described below. An important feature of the parallel elastic component is that these structures provide a resistive force as the muscle is passively lengthened above a critical length. However, when a muscle contracts and generates force, the tendon (series elastic component) will stretch, allowing the muscle fibres and parallel elastic component to shorten. The parallel elastic component will hold less force as the muscle fibres shorten and the tendon elongates during an isometric (fixed end) contraction.
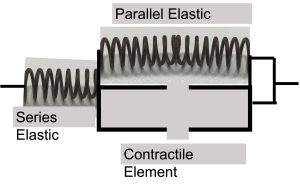
Fascicles are Bundles of Muscle Fibres
Muscle fibres are bound together in bundles called fascicles. Fascicles are surrounded by connective tissue called perimysium. Motor units are a collection of myocytes innervated by a single motoneuron (see Figure 4-2). Each motor unit may contain hundreds of myocytes and these cells are distributed across several fascicles while each fascicle will contain myocytes from several motor units.
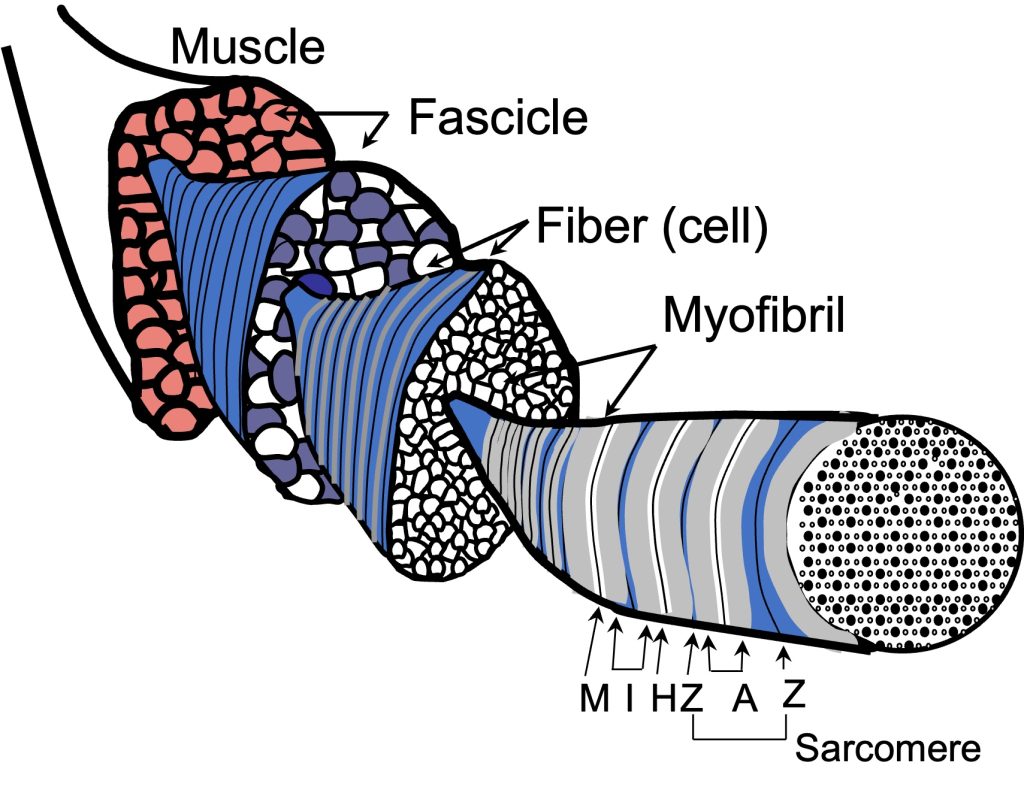
Shapes of Muscle Relate to Function
Skeletal muscles have a variety of shapes to accommodate a variety of functions. There are 3 primary categories of muscle shape:
- Parallel fibred
- Fusiform
- Pinnate
- Unipinnate
- Bipinnate
- Multipinnate
In general, muscle fibres are either oriented in line with the line of action of the muscle (parallel fibred and fusiform), or the fibres are aligned at some angle with respect to the direction of the line of action of the muscle (pinnate). Pinnate muscle can be unipinnate where all fibres are at a similar angle, bipinnate where the fibres are oriented in two primary directions or multipinnate where fibres are oriented in more than two directions. These muscle shapes are illustrated in Figure 4-3. Each shape of muscle has some advantages and some disadvantages, as will be described below.
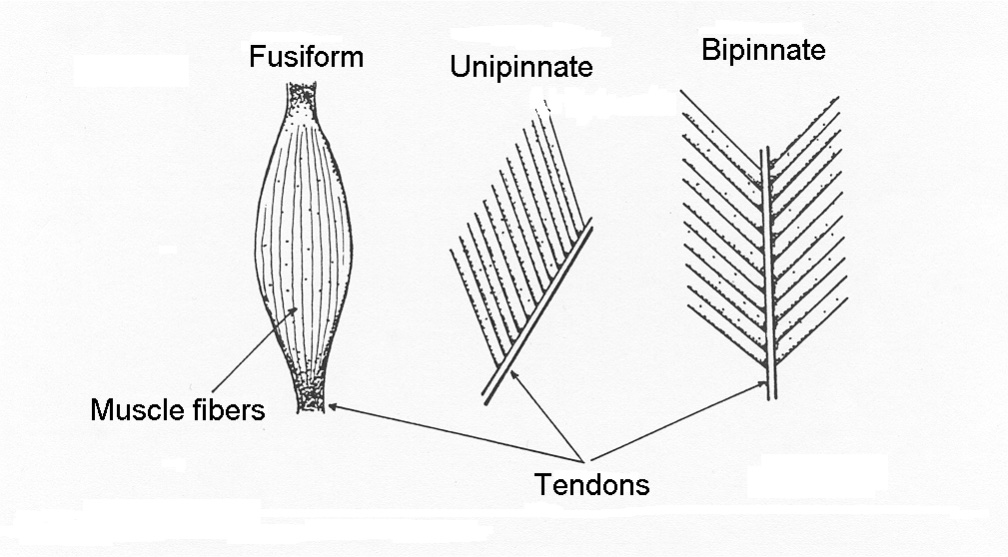
Parallel Fibred Muscle:
myofibrils and in many cases, more fibres arranged in series. Length of the muscle provides the advantage of a greater range of shortening and the potential for higher absolute velocities of shortening. The strap muscles of the neck are also parallel fibred muscles.
Fusiform Muscle:
fusiform muscle is one that is thick in the middle but narrows towards each end. The muscle fibres of a fusiform muscle tend to be aligned with the direction of pull of the whole muscle. This shape of muscle is of benefit for having a relatively large cross-sectional area but can originate and insert in relatively confined areas. An example of a fusiform muscle is the biceps brachii. Individual muscle fibres of a fusiform muscle are shaped the same as the whole muscle: wider in the middle range but narrow towards the ends. The muscle cells achieve this shape by terminating some myofibrils well before they reach the ends of the cell. The maximum isometric force that the muscle can generate is proportional to the cross-sectional area near the middle of the muscle and some fibres rely on lateral transfer of force. Lateral transfer of force is the transfer of force to adjacent structures (connective tissue) and also allows length change of all myocytes within a muscle even when only a few motor units are activated.
Pinnate Muscle:
A pinnate muscle (also called pennate) is a muscle that has the individual cells oriented in a direction that is at an angle to the direction of force application. The medial and lateral gastrocnemius muscles are examples of pinnate muscles. The deltoid is a multipinnate muscle, because the fibres are oriented in several directions. Pinnation, a term that derives from the Greek “pinne” or featherlike, allows packing a greater cross-sectional area into a given volume of muscle. A greater cross-sectional area allows greater force production by the muscle. However, individual fibres are shorter, so the property that allows greater force results in compromise of slower maximal absolute shortening velocity and possibly decreased range of motion.
Retinacula Decrease the Moment Arm of Skeletal Muscle
The tendons of some muscles are attached to bone at some distance from the joint, but the tendon is held close to the joint by a fibrous band called a retinaculum (the plural of retinaculum is retinacula). This is true in particular for muscles of the wrist and ankle. One feature of this arrangement is that it allows more individual muscles to operate across a joint, but the muscles can be attached at some distance from the joint. A retinaculum offers the advantage of decreasing the effective moment arm of a muscle (see Figure 4-4). This advantage allows a greater angular range of motion with less shortening of the muscle. Another advantage of the retinaculum is that a greater angular velocity of movement can be achieved for a given velocity of shortening. The moment generated by a muscle is the product of the force times the motor arm distance. For this reason, a retinaculum effectively decreases the potential maximum moment that the muscle in question can generate.
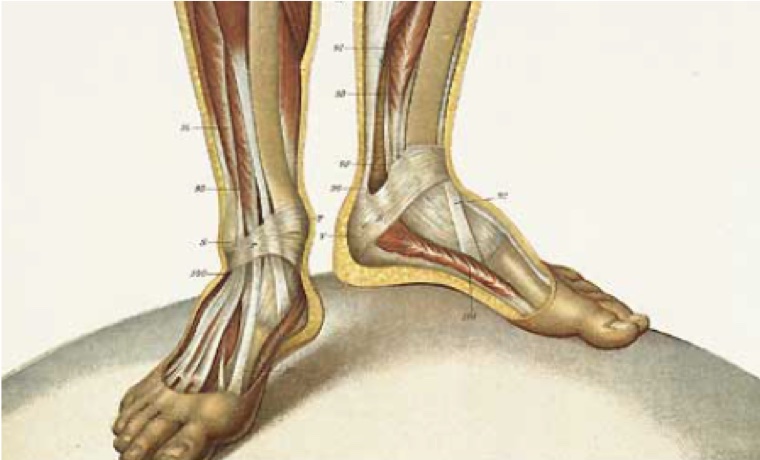
Connective Tissue in Skeletal Muscle
Connective tissue in skeletal muscle exists at five levels: around each cell, around bundles of cells called fascicles, around each whole muscle and connecting muscle to bone . These five levels of connective tissue are called:
Connective tissue is a fibrous mesh of collagen, elastin and scattered cells called fibroblasts. Connective tissue serves several functions: it gives tissue form and guides initial formation as well as regeneration following injury. Connective tissue provides a space for blood vessels to penetrate the tissue and forms a protective sheath around muscle. The tendon is also composed of connective tissue and serves an important role in skeletal muscle function, as discussed below.
Endomysium
The endomysium is a thin layer of connective tissue around each myocyte. Individual fibrils of collagen, which run in all directions, are relatively thin (60 to 120 nm). The endomysium provides a protective layer around each cell, outside of the basement membrane. The endomysium and basement membrane provide a cylinder in which muscle regeneration can occur during recovery from muscle damage.
Perimysium
The perimysium is a thicker layer of connective tissue that divides the muscle into fascicles. Arterioles and nerve branches use the perimysium to distribute throughout the muscle.
Epimysium
The epimysium, also known as fascia, envelopes each muscle in a thick layer of connective tissue. Collagen and elastin provide protection for the muscle while still permitting the shape changes needed to accommodate muscle shortening and elongation.
Tendons
Tendons are also composed of connective tissue, primarily collagen with small amounts of elastin and proteoglycans. Tendons connect skeletal muscles to bone, and in most cases there is tendon at both ends of a skeletal muscle. In some cases, the tendon is relatively compliant and in other cases there is a stiff tendon. Tendons can occupy much of the muscle-tendon length or they can be relatively obscure. It is important to realize that a muscle can stretch the tendon in series with that muscle and the magnitude of stretch depends on the tendon stiffness and the force of muscle contraction. The important realization from this relationship is that when a muscle contracts without changing joint angle (at either end of the muscle if it is a two-joint muscle), the muscle fibres can still shorten to accommodate the stretch of the tendon. This means the contraction is not truly isometric, and should be referred to as a fixed-end contraction.
Aponeurosis
The aponeurosis is a connective tissue surface to which myocytes of pinnate muscles are attached. In this respect, aponeurosis is like tendon. However, the function of the aponeurosis is very different from the function of the tendon. The aponeurosis must be very compliant. It accommodates the shape change of the individual muscle fibres as they shorten and become wider or elongate and become narrow. The surface of the aponeurosis must allow individual fibres to remain tightly attached to neighboring myocytes while these shape changes occur. The aponeurosis also assists with transfer of force to the tendon. However, lateral transfer of force also helps in this matter (Monti et al. 1999). Lateral transfer of force relates to the ability of a myocyte to transfer force to adjacent cells along the length of the fibre.
Blood Supply of Skeletal Muscle
Like all tissues of the body, each muscle will receive blood flow through 1 or 2 arteries and this/these arteries will go through several branchings and become the following vessels:
- Small artieries
- Arterioles
- Capillaries
- Venules
- Small veins
- veins
Vascular arborization
skeletal muscle is important for several reasons: providing oxygen and substrates for metabolism, removing products of metabolism and excess heat, and communicating with and from muscle by hormonal and cytokine transportation. Most muscles in the human body have a primary artery providing all of the necessary blood flow. This primary artery will divide several times, with each division resulting in two vessels of smaller radius but increased total cross-sectional area. By this division, the speed of flow of blood and the resistance to flow will both decrease. Blood travels sequentially to arterioles, capillaries, venules and veins. This sequence is like the main water supply to your house or apartment, branching to provide water to the many sites inside that need it, (sinks, toilets, showers etc) and the drains combining to form a single waste water exit to the sewer system are like the veins of a muscle.
Arteries:
Arteries are thick-walled elastic vessels that conduct the blood from the heart to the target tissue (muscles in this case). A primary artery (sometimes two) will enter each muscle, and divide several times, each time decreasing the diameter of the branches relative to the vessel before the branch. Eventually, arteries give way to arterioles, the primary resistance vessels of the vascular system. Vasoconstriction of arteries and arterioles increases the resistance to blood flow and vasodilation of these vessels decreases resistance allowing higher blood flow.
Arterioles:
Arterioles are smaller than arteries, but have relatively thicker walls, composed of smooth muscle layers and connective tissue. Arterioles will branch a few times before delivering blood to the capillaries. Arterioles are considered important for regulating the distribution of blood flow because they have the potential for very strong constriction.
Capillaries:
The purpose of the vascular system is to provide a means for delivering oxygen, substrates, hormones and cytokines to the various tissues and to remove substances that would otherwise accumulate in the extracellular space. It is in the that this function is achieved. Capillaries are thin-walled vessels that allow transport of these substances to and from the extracellular space within the tissues. The walls of capillaries are composed of a single layer of endothelial cells. Capillaries are barely large enough for red cells to squeeze in. The length of capillaries is about 1 mm on average. Exchange of substances between the vascular space and the extracellular space occurs by transport through or between the endothelial cells. In spite of being so short, the total number of capillaries in the body, when laid end-to-end would stretch all the way around the Earth (~40000km).
Venules:
Capillaries converge to form venules which are small enough and have thin enough walls that some exchange of gas, ions and small molecules can still occur here. However, the primary function of these vessels is directing blood flow from the tissues to the veins.
Veins:
Venules converge to form veins. Veins are the vessels that return blood flow to the heart from the periphery. Veins also serve as a large reservoir of blood, containing about 60% of the total blood volume. The walls of veins have smooth muscle in them, allowing to increase venous pressure. A higher venous pressure will allow a greater pressure difference between the veins and the heart, increasing blood return to the heart. Venous return is a primary determinant of cardiac output.
Lymph Vessels
In passing through tissue, blood will typically lose a small volume of fluid. To account for fluid distribution over time, this volume must be returned to the circulation. This return is accomplished by the lymphatic system, a series of thin-walled vessels that parallel the venous vascular system, emptying the contents into the subclavian veins.
The Motor Unit Organization of Skeletal Muscle
Structure and Distribution of Motor Units
A motor unit is a single motoneuron and all of the myocyte innervated by that motoneuron. Each muscle will have a number of neurons with soma in the ventral horn of the spinal cord. Each motoneuron soma in the ventral horn of the spinal cord gives rise to a single axon that exits the spinal cord to the ventral root and travels in the corresponding spinal nerve to the respective muscle. As the axon approaches the muscle, and within the muscle, it divides multiple times. Each myocyte is innervated by a single axon. The number of nerve branches, and therefore the number of myocytes innervated for each motor unit, depends on several factors: size and function of the muscle, as well as the fibre-type of the muscle fibres innervated. All myocytes of a given motor unit are the same fibre-type. Individual myocytes associated with a given motor unit are distributed through a region of a muscle. This means that when only a few motor units are active, it is highly unlikely that several adjacent myocytes are active.
Innervation and Neuromuscular Junctions
Each myocyte receives innervation from a single motoneuron. At the axon terminus, a specialized structure permits transmission of the activation from the axon terminus to the myocyte membrane. This transfer of activation occurs via chemical communication resulting from an action potential arriving in the nerve terminal. The neuromuscular junction includes the nerve terminus, the muscle membrane immediately under the nerve terminus and the gap between these two. Figure 4-5 illustrates a neuromuscular junction.
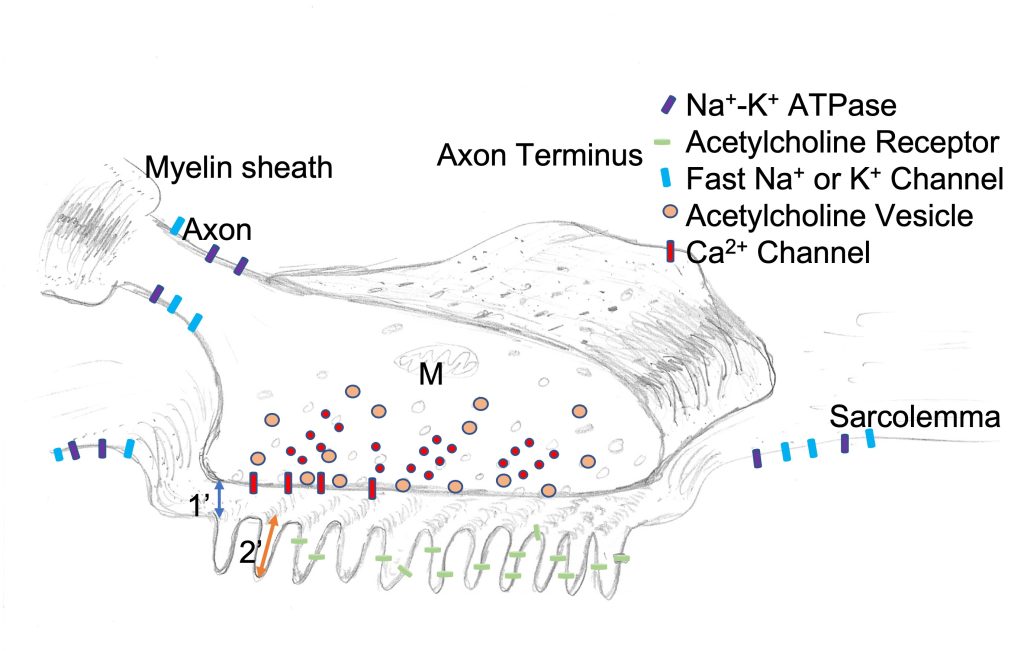
The nerve terminal is a specialized neural structure with membrane vesicles containing acetylcholine and the neurolemma contains special membrane proteins that allow tethering, docking and fusion of membrane vesicles with the nerve terminus membrane. In addition to these proteins in the membrane of the vesicles there are special proteins in the nerve terminal membrane that complement the special membrane proteins of the vesicles and participate in the process of docking and fusion. The nerve terminal also contains mitochondria to provide energy for the active processes that go on here. The membrane of the nerve terminus contains several pumps, channels and binding proteins. In addition to the fast Na+ channels and K+ channels, a predominant protein in the nerve terminus membrane is a Ca2+ channel. This channel opens briefly when an action potential arrives at the nerve terminus. The resulting puff of Ca2+ that enters through the Ca2+ channels will cause the local [Ca2+] to increase. This increased concentration results in binding of Ca2+ to one of the specialized proteins associated with docked vesicles and results in fusion of some of the docked vesicles and the consequent release of acetyl choline into the synaptic cleft.
The End Plate
The muscle membrane under the nerve terminus and the cytoplasm immediately under that membrane have specialized structures that serve the purpose of receiving the signal from the motor nerve and transmitting that signal to a muscle membrane, resulting in an action potential. The end-plate is illustrated in Figure 4-5. A key feature that identifies the end-plate, besides the obvious nerve terminal over this region, is the deep infolding of the sarcolemma and the presence of acetylcholine receptors in this membrane. These secondary s increase the surface area of the end-plate, providing more area for the acetylcholine receptors and decreasing the chance that acetylcholine will diffuse away from the end-plate region. The primary feature of the end-plate is the high concentration of acetylcholine receptors. Other areas of the sarcolemma do not have acetylcholine receptors, unless innervation for that muscle fibre is lost (Axelsson and Thesleff 1959). Denervation results in loss of activation, and activation is necessary to inhibit synthesis of acetylcholine receptors. Normally, myotrophic factors released from the motoneuron will overcome this inhibition for nuclei close to the neuromuscular junction. This is why acetylcholine receptors are normally located only at the neuromuscular junction.
Motor Unit Size
We know that each muscle fibre is innervated by a single axon, and that each motoneuron innervates many muscle fibres. The actual number of myocytes innervated by a given motoneuron varies considerably in the muscles distributed throughout the body. Note that muscles with fine motor control have few myocytes per motoneuron whereas muscles with less demand for fine motor control have a large number of muscle fibres per motor neuron (see Table 4-1).
How do we Know How Many Motor Units per Muscle?
There are various methods for estimating the number of motor units in a given muscle. The most direct is to count the number of apparent motor nerves entering a muscle. This gives only an estimate since the count depends on identifying motoneurons and not counting the motoneurons. This approach requires counting the large axons to exclude sensory neurons. Estimates of the number of motor units per muscle are given in Table 4-1 for a few human muscles. In this Table, the fibres/unit represents an average but the actual number of fibres per unit in a given muscle will vary considerably because every muscle has small and large motor units.
Muscle |
No. of Motor Units |
Muscle fibres/unit |
---|---|---|
First dorsal interosseous |
119 |
340 |
Brachioradialis |
333 |
>410 |
Tibialis anterior |
445 |
562 |
Gastrocnemius (med) |
579 |
1934 |
Masseter |
1452 |
640 |
Temporalis |
1331 |
936 |
Flexor digiti minimi |
130 |
108 |
Source: MacIntosh, Gardiner and McComas, 2006
An alternative approach to estimate the number of motor units is to stimulate the motor nerve with a progressively larger pulse (beginning with a very low voltage and gradually increasing the intensity for sequential contractions) while measuring the (McNeil et al. 2005). If the voltage begins low enough to activate a single motor unit and each increment adds only one motor unit, then motor units can be counted. A small increase in voltage that does not result in an increase in EMG amplitude is a null result, no additional motor unit was activated.
Fibre-Types in Skeletal Muscle
Typically, individual motor units can be identified as specific fibre-types. In human muscles these fibre types can be separated into fast and slow, but the fast fibre type has two subtypes. The classification of fibre type is either by contractile and metabolic factors or by myosin isoform identification. Although we classify motor units according to these fibre types, in reality, individual motor units can have properties that are intermediate between the classic fibre types. This occurs because some fibres can have two different isoforms of myosin heavy chain heavy chain, the major protein that distinguishes the fibre-types. Table 4-2 presents some typical contractile and metabolic properties that are associated with the classic fibre types.
Property |
Type I Slow-twitch |
Type IIa Fast-twitch |
Type IIx Fast-twitch |
---|---|---|---|
Twitch time |
long |
short |
short |
Twitch amplitude |
small |
large |
largest |
Maximal velocity of shortening |
low |
high |
highest |
Sag |
no |
yes |
yes |
Aerobic capacity |
high |
high |
low |
Glycolytic capacity |
low |
high |
high |
Relaxation rate |
slow |
fast |
fast |
Mitochondrial density |
high |
high |
low |
Myoglobin concentration |
high |
high |
low |
Fatigability |
low |
low |
high |
Contractile Properties and Classification of Fibre Types:
The initial classification of motor units into fibre-types was presented by Burke et al. (1973). These investigators identified three distinct categories of motor units in cat muscle according to contractile properties. They evaluated several possible contractile properties, but only a few of these had distinct characteristics that allowed separation of the motor units into three types: fast-twitch, fatigable, fast-twitch, fatigue resistant and slow-twitch. The key contractile properties included the following: force, twitch time, and fatigue index. The speed of the twitch contraction also contributes to fusion and the force-frequency relationship (described further in Chapter 5 on contractile properties). The separation of motor units into fibre-types is less distinct in human muscle than in cat, partly because of the presence of more hybrid fibres (see below).
A single stimulus to a motoneuron will result in propagation of a single action potential on the axon of the motoneuron. This single action potential will follow all branches of the motoneuron and transfer a single activation to each muscle fibre associated with that motor unit. The subsequent contraction of all these muscle fibres is called a twitch. A twitch contraction will have a rise and fall of force as illustrated in figure 4-6. The amplitude of the twitch is dependent on the average fibre size and how many fibres are innervated by that motoneuron. Typically, the force of a twitch is 15-20% of the force of a high frequency stimulation (tetanic contraction). Small motor units (type I) will result in small force and large motor units (type II) will result in large force. The duration of a twitch is also dependent on the fibre type. Two classifications of fibre type can be identified by twitch contraction time or time to peak force of a twitch: fast and slow (also known as type II and type I, respectively). It is important to realize that although within a species there is a difference in contraction time between fibre types, there is also a difference between species for any fibre type. The fast-twitch motor unit of a rat has a contraction time of 12-16 ms but the fast-fast-twitch motor unit of a human has a contraction time of 30-40 ms.
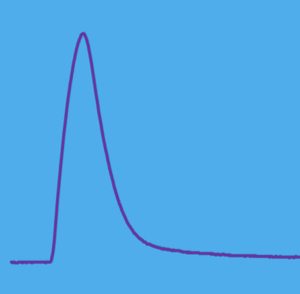
Myosin Isoforms and Classification of Fibre Types
There are minor differences in the amino acid sequence of the S1 segment that give the myosin head different kinetics. These differences in amino acid sequence are recognized as different isoforms of myosin. There are at least 9 different myosin isoforms recognized, but in adult human muscle there are only 3: type I, type IIa and type IIx. These myosin isoforms correspond to slow-twitch, fast oxidative (fast-twitch fatigue-resistant) and fast glycolytic (fast, fatiguable) respectively. Type I and IIa have high resistnce to fatigue and type IIx fatigues easily. A fatigue index can be calculated, based on the reduction in force observed over a fixed duration of time (usually, 0 to 2 or 60 min). This fatigue index is illustrated in Figure 4-7.
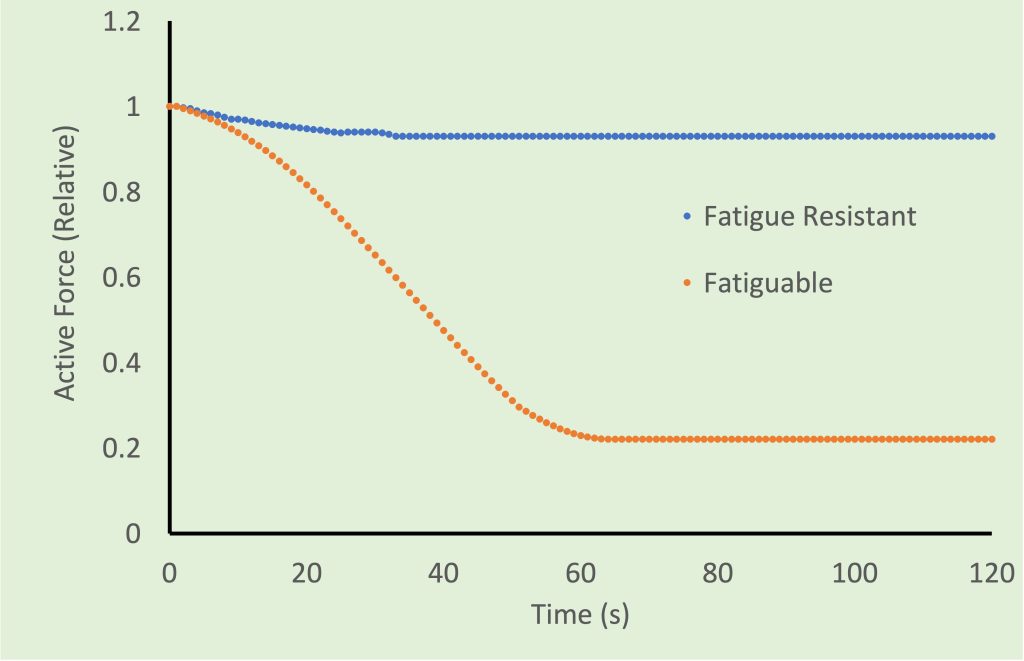
It should be pointed out that myosin isoform compliment is slightly different between species. Histochemical and contractile assessment identifies three fibre-types. However, there are 4 myosin isoforms, corresponding to type I fibre, type IIa, type IIb and type IIx. Type IIx was not identified until 1989 (Termin et al. 1989), so prior to that, it was thought that human muscle contained type IIb myosin, corresponding with the fast-twitch fatiguable contractile properties, similar to rat and cat muscle. However, identification of type IIx myosin in human muscle (initially referred to as IId), and the realization that type IIb does not exist in human muscle is an important fact.
Sag is a unique feature of fast-twitch motor units. When motor unit stimulation results in incompletely fused tetanic contraction, the oscillations in force reach a peak early in the repetitions followed by a subsequent decrease in peak values (see Figure 4-8). This decrease in force, or sag, is recognized as a property of type II motor units. Similar stimulation in Type I motor units results in a that continues to rise to a plateau with no decrease in force during the incompletely fused contraction.
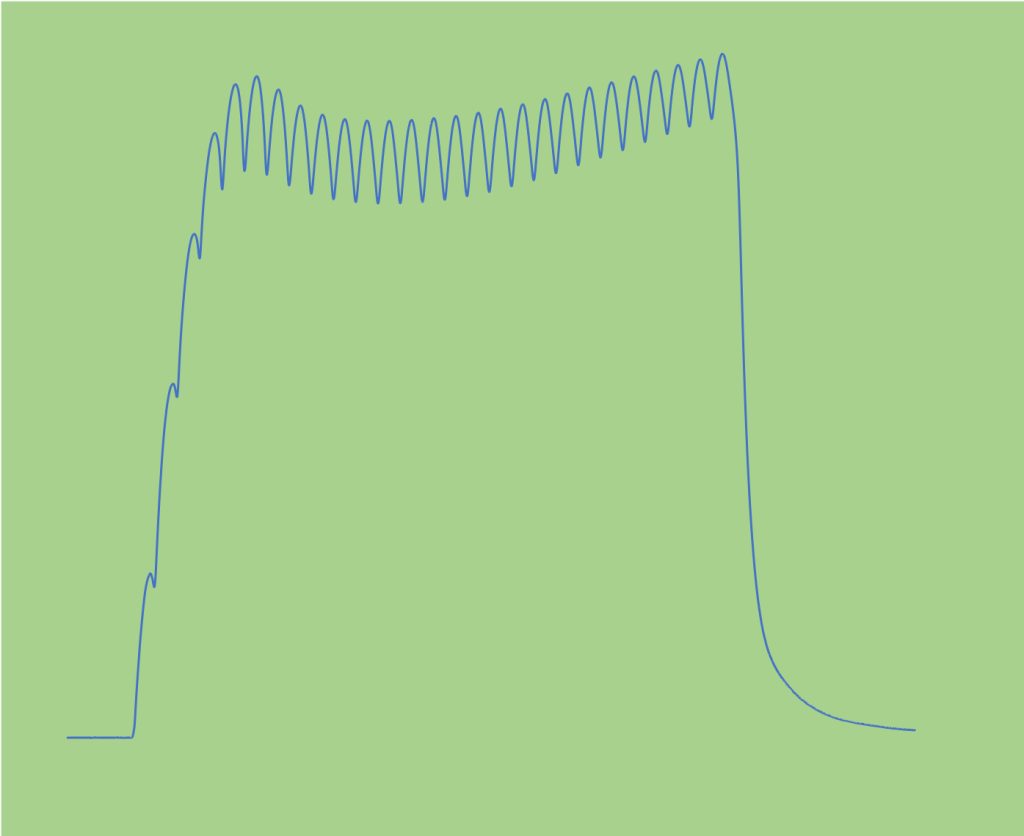
Although not considered by Burke and colleagues when they were evaluating contractile properties of the motor units, the force-frequency relationship can also be used to discriminate between motor unit fibre types. The force-frequency relationship has a sigmoidal shape (see Figure 4-9). The frequency of stimulation that gives the half-maximal force is dependent on the and relaxation times. A slow motor unit will achieve half-maximal force at a lower frequency. A fast-twitch motor unit will achieve half-maximal force a higher frequency. This fibre-type difference is illustrated in Figure 4-9. Higher and lower here are relative and dependent on species. Generally speaking, small animals have muscles that contract on a shorter time-scale while large mammals have muscles that contract on a longer time-scale.
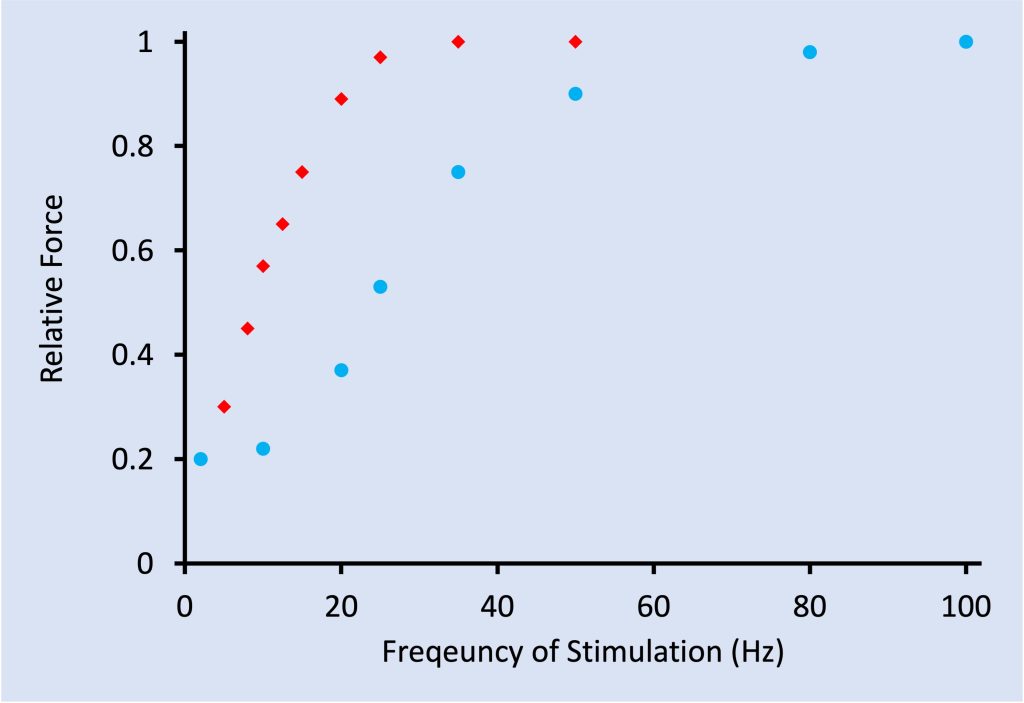
Hybrid Fibres
Some muscle fibres contain more than one myosin isoform. These are called hybrid fibres. The presence of hybrid fibres is a factor that blurs the distinction of fibre types by contractile assessment. Considering the presence of hybrid fibres, it has been suggested that fibre types should be represented by a spectrum rather than distinct fibre types.
Spectrum of Skeletal Muscle Fibre-Types:
Type I ↔Type I:IIa ↔ Type IIa ↔ Type IIa:IIx ↔ Type IIx
Myosin Light Chains
Contractile properties of motor units are also affected by isoforms of the light chains of myosin, which are attached to the myosin head (S1 segment). There are two classes of light chain: essential and regulatory. The regulatory light chain plays an important role in regulating the response to calcium sensitivity. Phosphorylation of the regulatory light chains improves calcium sensitivity (Persechini et al. 1985). These light chains are phosphorylated in response to high concentrations of Ca2+. Calmodulin is a small protein in the muscle cell, which has the capacity to bind Ca2+. When Ca2+ binds to calmodulin, this complex activates MLCK, an enzyme that phosphorylates the regulatory light chain of myosin. Phosphorylation of the regulatory light chains make the myosin head head more mobile (Levine et al. 1995). The myosin head tends to swing away from the backbone of the thick filament, bringing it closer to the actin. The proximity of the myosin head to the actin improves the probability for actin-myosin binding and crossbridge formation. The consequences of this increased mobility of the myosin heads are described in more detail in chapter 13 on history of muscle contraction.
Metabolic Relationships with Fibre-types
Red skeletal muscle fibres are associated with high capacity whereas white fibres have low aerobic capacity, but high capability. The red color is dependent on the presence of myoglobin, a protein similar to haemoglobin, but present in myocytes. You can probably guess that fatigue resistance relates to aerobic capacity, so slow-twitch and fast-twitch fatigue resistant motor units are red. White fibres are fatiguable. A high concentration of myoglobin is also associated with a high relative volume of mitochondria, which contributes to the high aerobic capacity of these red fibres. Mitochondria contain the enzymes responsible for aerobic replenishment of ATP. One of these enzymes, citrate synthase, has often been used to identify muscle with a high oxidative capacity.
Histochemical Identification of Fibre-types and Structural Differences
Historically, fibre-types were identified by histological treatments and staining. Histology is the study of microscopic tissue. Thin sequential slices of a muscle or biopsy of a muscle were preincubated in solutions of different then stained and inspected under a microscope. The pH of the solution affected the ability of specific isoforms of the myosin to lose or retain function. Subsequently, the slice of muscle was treated with a stain that developed only in fibres that preserved their ability to hydrolyze . As the ATP was split, the section of the slice of fibre got darker. By comparison of sequential slices of the muscle, individual fibres could be identified and classified as type I, type IIa or type IIb. In some cases, a fourth type was identified, and it was called type IIc. Only by analysis of myosin isoforms was it later demonstrated that fibres identified as Type IIc were actually hybrid fibres (type I-IIa). With the advent of fluorescence microscopy, and development of antibodies, it was further discovered that human muscle does not contain Type IIb myosin. Fibres thought to be Type IIb were actually Type IIx. There is some confusion in the research literature as this myosin isoform is sometime referred to as type IId. Histological determination of fibre type is based on myosin isoform. It is generally considered that the myosin isoforms also correspond with contractile properties. In human muscle the following myosin isoforms correspond to fibre types determined by contractile properties: type I is slow-twitch, type IIa is fast-twitch fatigue resistant and type IIx is fast-fast-twitch, fatiguable.
Sensory Receptors in Muscle
Before we consider the subcellular structures of muscle cells, the structures in muscle that detect various parameters will be presented. Sensory receptors in muscles are connected to the central nervous system by afferent neurons that travel in the peripheral nerve to close to the spinal cord where they depart from the motor nerves to enter the spinal cord via the dorsal roots. The cell bodies of these sensory neurons are located in the dorsal root bodies, just outside the spinal cord. Within the spinal cord, these sensory axons may synapse with other neurons at the same spinal level or travel up towards the brain. Motor control relies on input of some of these sensory receptors.
Muscle Spindle
Muscle spindles detect length in muscle. Muscle spindles are distributed through-out each muscle in varying density, depending on the need for length sensing. Muscles with fine motor control tend to have more spindles. The structure of a muscle spindle is illustrated in Figure 4-10.
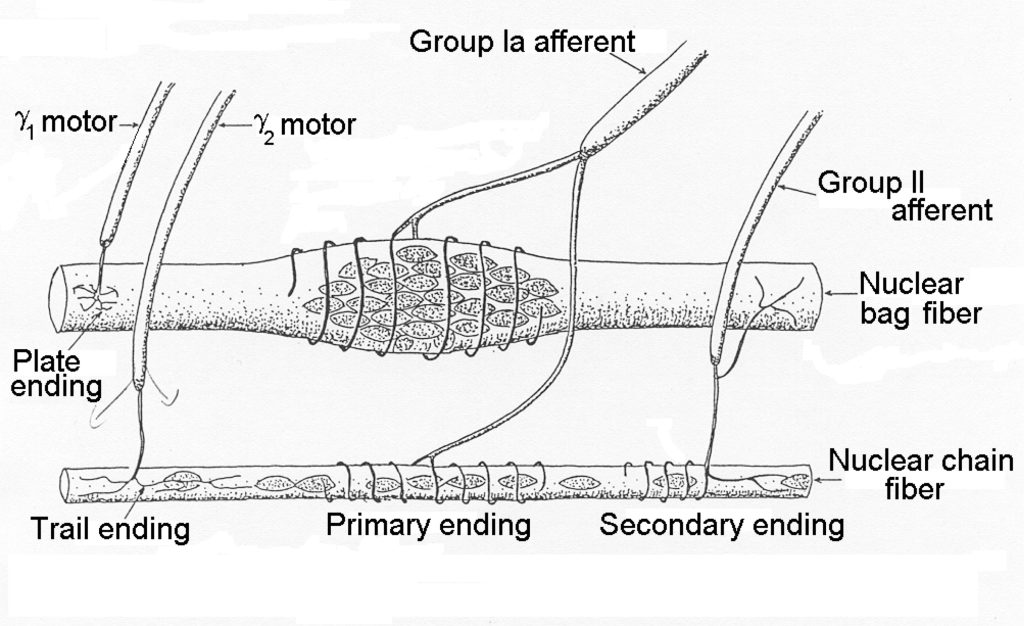
The cells within the spindle are called intrafusal fibres and they are either nuclear bag fibres or nuclear chain fibres. Typically, there are only one or two nuclear bag fibres and several nuclear chain fibres. It can be seen that the spindle is encapsulated and that several axons pierce the capsule. The efferent neurons are gamma motor neurons. The afferent neurons are Type Ia and Type IIa neurons that carry the signal from the intrafusal fibres to the central nervous system. The nuclear bag fibres are more important for sensing velocity of movement while the sensory chain fibres are activated in direct proportion to the length of the muscle. Afferent neurons from muscle spindles connect monosynaptically to alpha motor neurons of the same muscle, allowing a quick response to sudden stretch of the muscle. This is the response that is tested by the doctor’s hammer tap on the patellar tendon.
Golgi Tendon Organ
Within the tendons, there are small free nerve endings that weave between tendon fibrils. These nerve endings are activated in a frequency dependent manner when the tenson is under strain. In this way, the brain receives information about the force of contraction. In addition to providing the brain with this information, these sensory neurons connect to the same muscle’s alpha motor neurons , providing inhibitory input (Windhorst 2007).
Metaboreceptors
The term “metaboreceptor” refers to neural receptors that are sensitive to metabolic products in the interstitial space. Metabolic products include CO2, potassium, and H+. Metaboreceptor is used for a collection of nerve endings that are sensitive to specific chemicals. It is generally thought that these receptors are sensitive to a variety of things including: oxygen, hydrogen, carbon dioxide, potassium. It is important to realize that these chemically sensitive nerve endings are located in the extracellular space. They do not detect intracellular chemical disturbance. These receptors are important for regulation of the blood flow in muscles. The ability to detect chemicals was confirmed by noting that these neurons continue to fire after muscle ends when blood flow is occluded (Piepoli et al. 1995).. Releasing the occlusion results in decrease in firing rate.
Mechanoreceptors
There are a number of receptors in each muscle that are sensitive to motion. These are mechanoreceptors or ergoreceptors (Francis et al. 1999). Not much is known about these receptors, but they probably provided the central nervous system with information about change of motion or muscle use. They adapt quickly, so firing is initiated on first use of a muscle, but declines in spite of continued These receptors are not active when the muscle ends activity.
The Skeletal Muscle Cells
Skeletal muscle cells are also called fibres or myocytes. Each fibre typically spans the distance between proximal and distal tendons. However, some long muscles have fibres arranged in series, either with a band of connective tissue, called an inscription separating bundles of fibres in series, or staggered in such a way that separations cannot easily be detected. In such long muscles, individual fibres do not extend from end to end. Skeletal muscle fibres are typically 20 to 90 µm in diameter and vary in length from a few mm to ~12 cm, depending on which muscle we are referring to. Muscles of the human body vary in size, so it is not fair to give an average number of fibres per muscle. Needless to say, the number can vary from a few thousand to several million. Although the number of fibres in a muscle can be different between individuals, the major difference between those with large muscles and those with small muscles is the diameter of individual fibres (MacDougall et al. 1984). Strength training leads to increased myocyte cross-sectional area, not an increase in number of myocytes (Alway et al. 1988).
Muscle Fibres (myocytes):
Skeletal muscle myocytes have most of the constituents that other cells of the body have: plasmalemma, nucleus (actually, several nuclei), mitochondria, endoplasmic reticulum, cytoskeleton. However, myocytes also have specialized structures associated with its special functions: contraction, force generation and mobility. Muscles have myofibrils containing contractile proteins actin and myosin as well as transverse tubules and sarcoplasmic reticulum.
Satellite Cells
Skeletal muscle also contains satellite cells, which are nonspecific cells, lying under the basement membrane of individual skeletal muscle cells. Considering that myocytes are multinucleated, it is difficult to distinguish myocyte nuclei and satellite cells, which are simple single cells containing very little cytoplasm, so they just look like myocyte nuclei. The only distinguishing feature is that satellite cells have a plasmalemma, in addition to a nuclear envelope whereas nuclei within the myocytes are surrounded only by a nuclear envelope. Satellite cells can undergo mitosis and some can become incorporated into the muscle cell during hypertrophy. This process keeps the number of nuclei per volume of muscle constant. When injury results in myocyte death, satellite cells can become myoblasts, and fuse to become new muscle cells. Since this process occurs within the basement membrane, this is a replacement process, not creation of new myocytes.
Subcellular Structure
Each myocytes is surrounded by a sarcolemma and contains a standard selection of intracellular components. In each myocyte, there will be several nuclei, a mitochondrial reticulum, sarcoplasmic reticulum, myofibrils and glycogen granules.
Sarcolemma
The sarcolemma is the membrane plus basement membrane of skeletal muscle myocytes. A unique feature of the sarcolemma is that there are periodic invaginations of the membrane called transverse tubules that allow conduction of the action potential into the cell. These structures are described below.
Plasmalemma
Like all cells of the body, the muscle fibre has a lipid bilayer cell membrane, referred to as plasmalemma. The combined plasmalemma and basement membrane of skeletal muscle is called the sarcolemma. Embedded in the sarcolemma are a variety of proteins: pumps, channels, receptors, enzymes and structural proteins that serve to strengthen the cell membrane and transfer force across the cell membrane. A unique feature of skeletal muscle is that adjacent cells are linked at recurring structures called costameres (see below).
Basement Membrane
The basement membrane, a fine mesh of glycoproteins, encircles each muscle fibre. It serves several functions. The basement membrane serves to attach the myocyte to the endomysium. At the neuromuscular junction the basement membrane tethers the enzyme acetylcholinesterase which is important for termination of activation of the myocyte. The basement membrane also serves as a scaffolding for myocyte regeneration in the event of muscle damage. The basement membrane is also thought to be important for guiding regenerating motor axon to the end-plate region of the myocyte and regulating the formation of a nerve terminal.
Transverse Tubules
A unique feature of skeletal muscle is the regular invagination of the plasmalemma, forming tubes that go deep into the myocyte. These membrane tubes serve to transmit the surface action potential to the myofibrils that are distributed across the cell. Transverse tubules (T-tubules) occur at regular intervals along the length of the myocyte at what is referred to as the triad (two terminal cisternae and one T-tubule; see Figure 4-11). Although at the surface, the T-tubules appear as wells that go deep into the myocyte, they actually encircle each myofibril, providing a location for activation at each end of the sarcomere (frog muscle) or near each junction (mammalian muscle). The specific location of the T-tubule in mammalian muscle appears to be fixed at 500 nm from the Z-disk (Brown et al. 1998). This puts the release of calcium near the middle of the thin filaments in mammalian muscle.
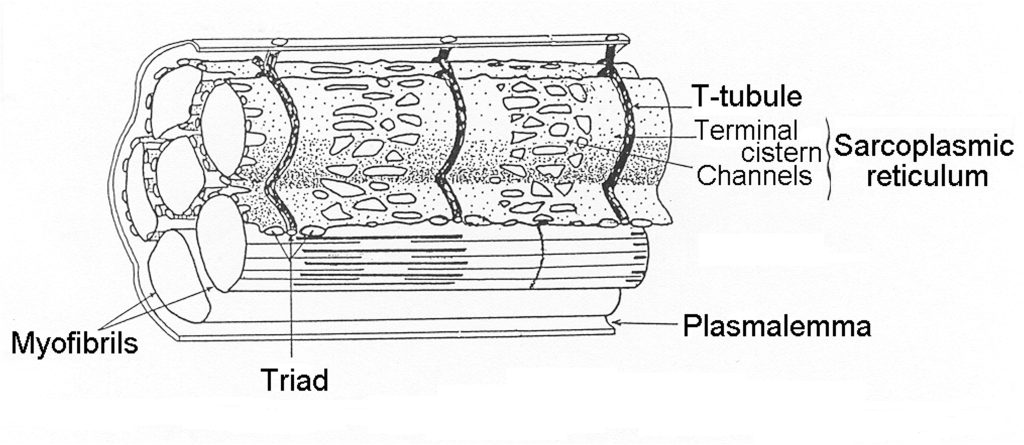
Cytoskeletal Proteins:
There are a number of proteins that serve the function of effectively transferring force across the membrane and of giving skeletal muscle shape and integrity. These proteins include a collection of proteins that connect the actins to the tendon through the folded plasmalemma at the ends of the myocyte, special proteins that connect to the dystroglycan complex and stiff proteins that keep things organized in the cell. These proteins are listed in Table 4-3
Connect Actin to Tendon |
Connecting & Intermediate Proteins |
Structural Proteins |
---|---|---|
actinin |
Dystrophin |
Actin |
Vinculin |
Desmin |
actinin |
Talin |
Laminin |
Spectrin |
Fibronectin |
Vimentin |
Myosin |
Capping protein |
Synemin |
Troponin (T,I,C) |
|
Myomesin |
Titin |
|
Creatine kinase |
Nebulin |
Myofibrils
Myofibrils are narrow tubes of contractile proteins, arranged in repeating units called sarcomeres. Each sarcomere (see Figure 4-12) spans the distance between Z-disks; a distance that can vary from about 1.6 µm to close to 4 µm as muscle changes length. Beyond 4.2 µm filament overlap no longer occurs and a sarcomere cannot generate active force through actin and myosin interaction. Each myofibril is about 1 µm in diameter and runs the full length of the muscle fibre, but can become narrow near the ends of a fusiform muscle. Titin and nebulin form a structural framework to align the filaments: actin and myosin in a highly structured myofilament lattice. Titin runs from the M-line to the Z-disk with 6 molecules of titin extending from the end of each thick filament. Nebulin runs along the thin filament, actin, and may serve as a template to limit the length of actin filaments.
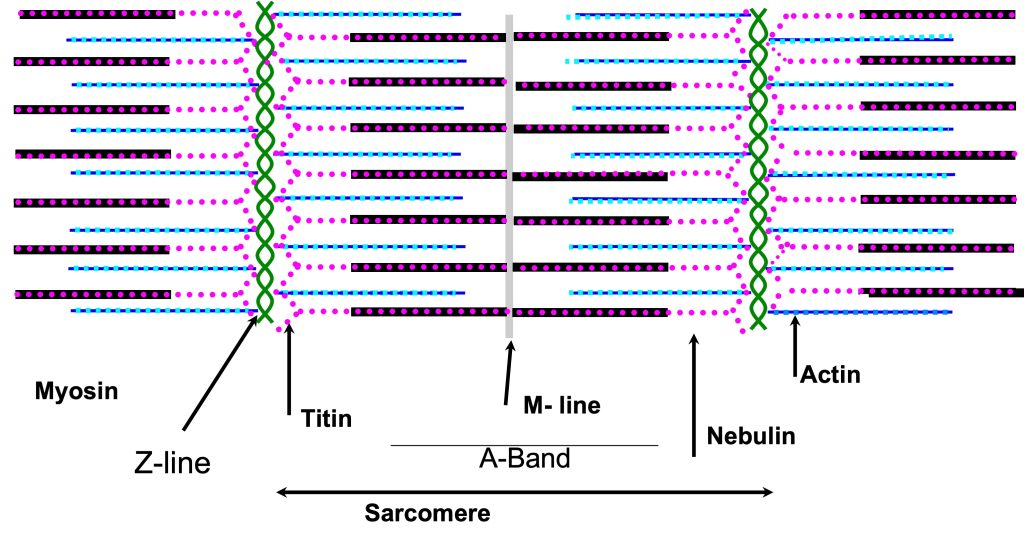
Sarcomeres: the Reason Skeletal Muscles are Striated
A sarcomere is defined by the myofibrillar unit from one Z-disk to the next (see Figure 4-12). The sarcomeres in adjacent myfibrils are connected by intermediate filaments collectively called the costamere. This connection allows the Z-disks in adjacent myofibrils to be aligned, The result is that skeletal muscle has a striped or striated pattern (see Figure 4-2 and 4-11) because the thick filament of adjacent myofibrils are lined up, giving a dark band across the myocyte. The sarcomere has been labeled for the pattern of dark and light regions of the myofibril. The m-line is the middle of the sarcomere, and the Z-line (or disk) is at each end of a sarcomere. The A-band is the dark region where the thick filaments are and the I band is where only thin filaments are present (on either side of the Z-disk). The H-zone is the middle section of the A-band where only thick filaments are present. As the sarcomere changes length, only the H and I bands change in magnitude.
Costameres Join the Myofibrils Together
There is a mesh of intermediate filaments that form the Z-disks of the myofibrils and extend between myofibrils, holding them together across the cell. This mesh is called the costamere. In fact, the intermediate filaments, including desmin, vimentin, synemin connect at the surface membrane to the dystroglycan complex, and extend into the endomysium. Adjacent cells are aligned such that sarcomeres line up not only across myofibrils within the myocyte, but between cells across the whole muscle. The result of this interconnection between myofibrils and cells with aligned sarcomeres is the striped or striated appearance characteristic of skeletal muscle.
M-Line Struts Join the Thick Filaments
The sarcomere is an amazing and complex structure that displays mirror symmetry across the middle of the sarcomere. The thick filaments which seem to be suspended in the middle of the sarcomere, share this mirror symmetry with a short bare middle region followed by rows of protruding myosin head heads on either side of the bare zone. The myosin heads can interact with actins when the muscle is activated. However, the thick filaments are not freely floating in the myofibril. They are attached through the middle by proteins at the M-line. The proteins that make up the M-line include myomesin, M-protein and creatine kinase. These proteins, along with titin which connects the thick filaments to the Z-disk, keep the thick filaments aligned in the middle of the sarcomere.
Mitochondrial Reticulum
Mitochondria serve an important function in the myocyte; they replenish by oxidative metabolism. Mitochondria are often depicted as jelly-bean shaped organelles in cells. This should not be construed as the only shape that mitochondria can have. In some cells, mitochondria are more stick-like and in skeletal muscle, particularly red skeletal muscle, the mitochondria form an elongated and apparently continuous reticulum. That is, a single cross-section of the cell does not reveal the extent of connection between mitochondria that otherwise appear to be in different regions of the cell. A careful analysis of three-dimensional structure of skeletal muscle reveals that a single mitochondrion can extend over a large volume of the muscle fibre. The proportion of muscle volume occupied by mitochondria is a function of the oxidative capacity of the muscle and can generally be related to muscle fibre-type (Alway 1991). Type I muscle fibres have about 6-9% of total volume occupied by mitochondria while Type IIa fibres have almost as much (4-7%). Type IIx fibres have the lowest volume of mitochondria with just 2-3% of their cell volume occupied by mitochondria. These values are in stark contrast with volumes of mitochondria in bumblebee flight muscle which has about 50% of total cell volume occupied by mitochondria.
Mitochondria can be described as existing in two designated regions of the myocyte: subsarcolemmal and intermyofibrillar. It is generally thought that subsarcolemmal mitochondria provide ATP for transport across the sarcolemma and intermyofibrillar mitochondria provide ATP for crossbridge function and Ca2+ transport into the sarcoplasmic reticulum.
The mitochondrial reticulum has an inner and outer membrane, each of which is a lipid bilayer, similar to the plasmalemma. The inner membrane is folded back and forth across the reticulum, forming protrusions into the middle, called cristae (See Figure 4-13). The surface area of the inner membrane is much greater than the surface area of the outer mitochondrial membrane. This two-membrane structure divides the mitochondria into compartments; there is an intermembrane space and the mitochondrial matrix. Like the plasmalemma, there are proteins embedded in the mitochondrial membranes. These proteins are ion channels and pumps as well as enzymes and transporters. Some of these proteins are presented in Table 4-4 with their location and function. The interior of the mitochondrial reticulum can be subdivided into the intermembrane space and the mitochondrial matrix. A recent review by Pfanner and colleagues presents a description of the location and function of a number of mitochondrial proteins (2019).
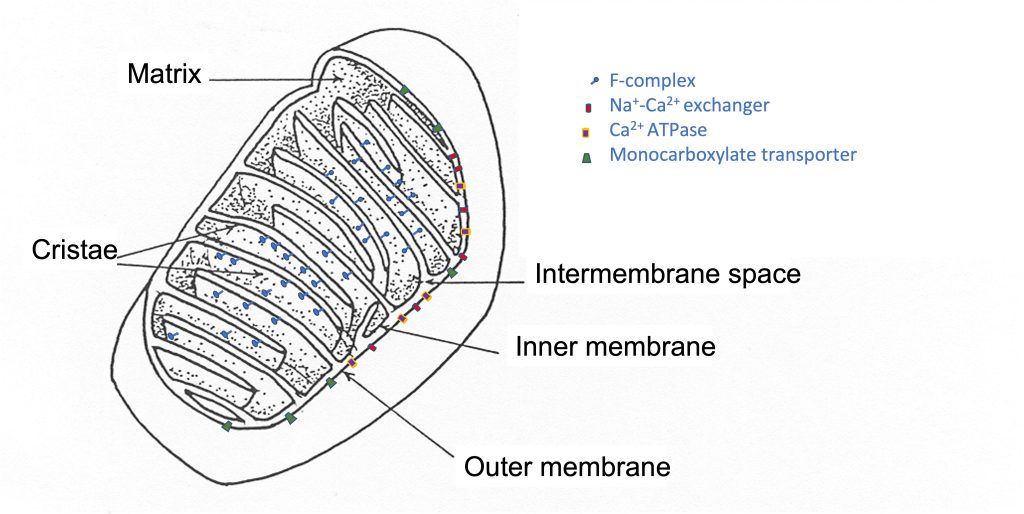
Outer Membrane |
Inner Membrane |
Matrix |
---|---|---|
ion transporters |
Complex I-IV |
TCA cycle enzymes |
Protein transporters |
F-Complex |
|
Monocarboxylate transporters |
Oxidative phosphorylation |
|
Source: MacIntosh et al., 2006, with permission.
Mitochondria also have several unique enzymes associated with metabolism. These enzymes include the enzymes associated with the Kreb Cycle which begins with combining acetyl CoA with oxaloacetate to form citric acid, which is subsequently converted back to oxaloacetate by a sequence of reactions that result in formation of Guanosine triphosphate, CO2 and NADH. See Chapter 6 for a list of these enzymes and the control of their function. In addition, the cytochromes associated with phosphorylation are present in the inner membrane, as is the very important F-complex, where the movement of H+ across the inner membrane drives the production of ATP. More detail about the function of these mitochondrial proteins is presented in Chapter 6.
Although most of the proteins expressed in the mitochondria are produced from DNA in the nucleus, some mitochondrial proteins are encoded by DNA in the mitochondria.
Sarcoplasmic Reticulum
The sarcoplasmic reticulum (SR) is a membrane-bound space encircling myofibrils, and in muscle cells is a specialized version of the endoplasmic reticulum found in most cells (See Figure 4-11). The SR serves the purpose of storing Ca2+ ions that will be released in response to arrival of an action potential in the t-tubules. The SR makes up approximately 2% of myocyte volume; a little more in fast-fast-twitch fibres and less in slow-slow-twitch. There are three essential proteins contained in the SR that are required for the function of the SR in excitation-contraction coupling (Shishmarev 2020). The ryanodine ryanodine receptor (RYR) is a Ca2+ channel protein that opens on activation of the myocyte, allowing Ca2+ to move through the SR membrane and into the cytoplasm of the sarcomere. Calsequestrin (CASQ) is a Ca2+-binding protein that buffers [Ca2+] in the SR and allows the SR to store large amounts of Ca2+ within a relatively small space. Finally, there is the Ca2+ pump, or Ca2+ ATPase which is important for transporting Ca2+ from the cytoplasm back to the lumen of the SR restoring the resting distribution of cytoplasmic [Ca2+], permitting relaxation to occur. This transport uses one molecule for each 2 Ca2+ ions transferred. The Ca2+ATPase is also known as sarcoendoplasmic reticulum ATPase (SERCA) and there are more than ten different isoforms of this pump in the body, but two isoforms are found in the SR of skeletal muscle simply referred to as SERCA1 (in fast-twitch fibres) and SERCA2 (in slow-twitch fibres).
Longitudinal Sarcoplasmic Reticulum
As the SR wraps around each myofibril it displays, what at first glance looks like a random pattern of windows along the surface through which you can see the surrounded myofibril. Upon closer inspection these windows or fenestrations appear with a somewhat repeatable pattern, particularly over the middle part of the sarcomere.
The SR is continuous (lacks fenestrations) where it joins with the t-tubules (terminal cisterna) to form the triad and is mostly continuous where the SR stretches over the z-disk (as shown in Figure 4-11). However, between those positions on the SR large irregular oval fenestrations appear. The exact purpose of these fenestrations is not fully understood. However, they result in reduction of volume of the SR and decreases the surface available for removal of calcium via the membrane bound SERCA pumps. In mammals a pair of triads are positioned in each sarcomere about 500 nm from the T-tubule. As a result, mammalian sarcomeres have twice as many t-tubules as amphibian muscle, and the RYR release Ca2+ directly over the actin and myosin myofilaments.
Terminal Cisternae
The longitudinal SR surrounding the myofibrils is bisected at regular intervals by the T-tubules. The SR in these sections exhibits several structural changes, and is referred to as the terminal cisternae although it remains continuous with the longitudinal SR. The luminal volume of the SR is greatly increased in the terminal cisternae and dotted with a series of membrane bound protein pores that give. In the terminal cisternae the SR is attached to the T-tubules through a series of membrane proteins imbedded in both the SR and T-tubules that are mechanically connected (See Figure 4-14). Dihydropyridine receptors (DHPR, also known as Cav1.1) are embedded in the t-tubule membrane and interact directly with a pair of RYRs embedded in the adjacent SR membrane through structures referred to as junctional feet, also referred to as the junctional membrane complex (JMC). There is a small gap between the DHPR’s in the t-tubules and RYR that are embedded in the membrane of the SR and it is into this space that calcium ions are released from the lumen of the SR into the cytosol of the myofibril through the RYR upon activation by action potential.
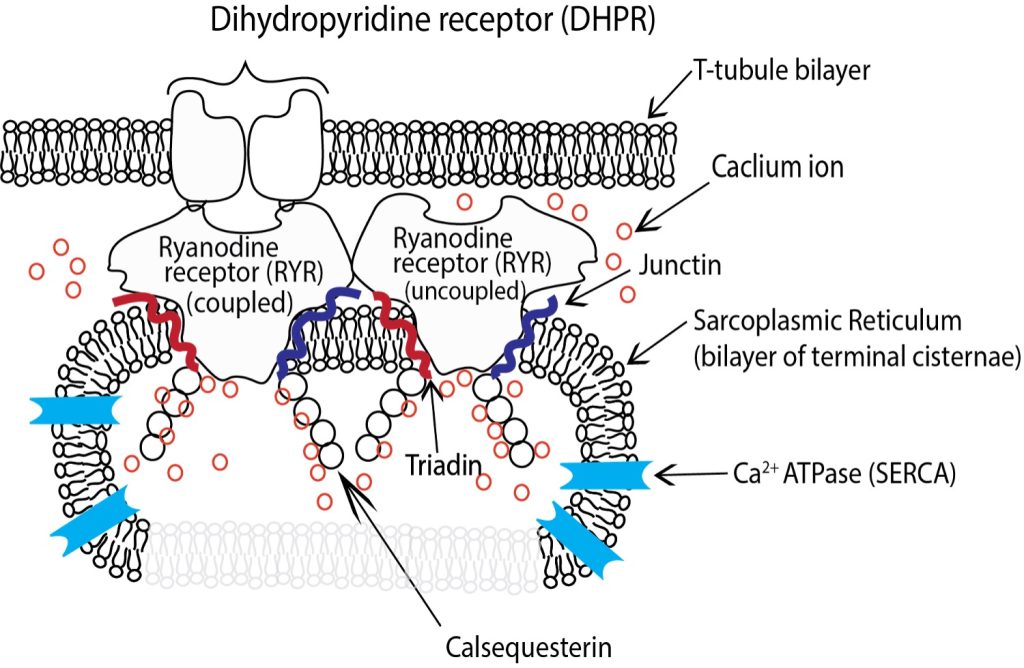
Junctional feet
The junctional feet in skeletal muscle are composed of several junctophilin proteins. Junctophilin 1 is the specific protein isoform that tethers the t-tubule to the DHPR and along with the II-III loop on the DHPR forms the electromechanical link that couples the DHPR to one RYR of each pair to control calcium release from the SR (Landstrom et al. 2014). It has been demonstrated that in situations of prolonged and high calcium concentration that the calcium activated protease calpain can be activated by the prolonged high concentrations of calcium and sever the JMH1 connection between the SR and t-tubules disrupting the triad (Murphy et al. 2013) dramatically reducing the ability of the SR to release calcium in response to further activation.
Accessory Proteins in the Sarcoplasmic Reticulum
Calsequestrin
Calsequestrin (CASQ) is a protein found in the lumen of the SR (See Figure 4-14) primarily in the junctional SR, near the transverse tubule. CASQ binds calcium and facilitates calcium storage at high concentrations. In humans there are two isoforms of CASQ. CASQ I is the isoform found in skeletal muscle and CASQ II is the isoform found in the heart. In skeletal muscle each CASQ molecule can bind up to 80 calcium ions depending upon how it is configured (Sanchez et al. 2012). At free [Ca 2+] below 100µM CASQ is found as a monomer, whereas at higher [Ca 2+] CASQ polymerises, first into dimers, then into a chainlike structure, binding more Ca2+ progressively. The binding of Ca2+ with CASQ allow the relatively small lumen of the SR to store calcium in concentrations up to 20 mM when the free concentration is about 1 mM.
Triadin & Junctin
In the lumen of the SR two additional proteins, triadin and junctin are associated with the RYR and play vital roles in Ca2+ handling and release (see Figure 4-14). Both junctin and triadin are concentrated on the luminal side of the SR membrane but have sections that extend to the cytosolic side. Triadin and junctin are located beside the RYR and both interact with CASQ to localize it near the luminal pore of the RYR.
Fine tuning of Calcium Release During Contraction
While the action potential is the primary signal that determines the open or closed state of the RYR. The open or closed state of the RYR is further modulated by the binding and interaction of ligands, both directly and through intermediary proteins such as ATP, ADP, CaM, CASQ, Triadin and Junctin. These ligands bind to either the cytosolic and luminal side of the RYR and alter its action. These interactions are complex, and our understanding of their influence on the behaviour of the RYR continues to evolve. The most common of these ligands, with the best-established influence on the opening probability of the RYR are listed in Table 4-5. While these ligands seldom override the direct control of the DHPR they do influence both the opening probability (OP) and duration which RYR are opened or closed during activation.
Increased OP |
Increase or decrease OP depending on concentration |
Decreased OP |
---|---|---|
ATP Caffeine S100A1 APOCaM CaMKII Triadin+CASQ |
FKBP12 Dantrolene Mg2+ Ruthenium red CaM+Ca2+ Junctin+CASQ |
Acronyms: adenosine; S100A1 is a modulating protein with Ca2+ binding properties; APOCaM is a primary form of calmodulin another Ca2+ binding molecule; CaMKII is calmodulin activated kinase II, an enzyme that is activated by binding calmodulin; CASQ is calsequestrin; DHPR II-II loop-STAC3 is the luminal loop of the dihydropyridine receptor with STAC3, a modulating protein, bound to it; cAMP cyclic adenosine monophosphate; FKBP12 is a small protein that is essential for ryanodine receptor function; CaM is calmodulin a small molecule that binds Ca2+ and in the bound state is responsible for activation of a number of enzymes.
Glycogen Granules
Glucose is stored in muscle in the form of polymers where individual glucose molecules are joined together with phosphate. The binding point of glucose is either 1-5 or 1-6 binding. This combination of binding allows branching of the glucose chain, which allows more glucose units in a given volume of cytoplasm.
Insulin Transporter Rafts: Glut4
The transporter molecule for moving glucose across the sarcolemma is known as GLUT4. This transporter is normally found inside the cell, where it cannot transport glucose. GLUT4 is stored in a form referred to as a raft, where several transporter molecules are bound together. Activation of the transporter by insulin or by increased intracellular [Ca2+] results in translocation of the raft to the sarcolemma where the GLUT4 molecules are inserted into the membrane. Here, they can transport glucose from the extracellular space into the myocyte.
Nuclei
The nucleus is an organelle that is the control centre of the cell; it controls protein expression and synthesis. Skeletal muscle myocytes, because they are so long, are multinucleated. The volume of the myocyte under the control of a single nucleus, which is called the myonuclear domain, is reasonably constant. As the cell grows by hypertrophy, nuclei are added and with atrophy, nuclei are removed (Ross et al. 2018). There is no evidence that skeletal muscle nuclei can divide to produce more nuclei. It is thought that satellite cells, located between the plasmalemma and the basement membrane divide and donate nuclei to the myocyte to keep the cell volume per nucleus relatively constant.
Membranes and Envelopes
Each nucleus is surrounded by a membrane called the nuclear envelope. The nucleus contains the chromosomes, which are made up of the genes for protein. A specialized structure within the nucleus is the nucleolus which regulates ribosome assembly.
DNA (deoxyribonucleic acid) is the molecule that contains the code for synthesis of proteins in the body. DNA is composed of nucleotides: cytosine, guanine, adenine and thymine.
Molecular Control and Protein Synthesis
Chapter 14 describes the control of protein from the perspective of cell signaling to turn on specific genes. This process serves to regulate which proteins are expressed in the cell as well as how much of the protein will be synthesized. The genes that encode the sequence of amino acids for a given protein are stored in the nucleus. Cell signaling activates the gene, causing transcription which forms a messenger RNA (mRNA). This mRNA is transported out of the nucleus to the ribosome where it serves as a template for protein. Newly synthesized proteins can be moved to where they are needed. Posttranslational regulation of proteins will alter their activity. This regulation can include adding phosphate group(s) to the protein, cleaving a portion or other modifications that allow change in the function of the protein.
Protein synthesis can occur to replace proteins that are already in the myocyte, to increase the amount of a protein or to change the isoform of the protein that is currently in the myocyte. Each protein in a cell has a typical time for which it “survives” in the cell. Protein turnover helps to keep the cell alive and functional by getting rid of old proteins, replacing them with newly synthesized molecules. The half-life of individual proteins (time over which half the molecules of that protein are replaced) varies considerably from days to years.
Proteins of Skeletal Muscle
There is a multitude of additional proteins found in skeletal muscle. The majority of these proteins can be categorized as follows: motor proteins, channels, pumps and transporters, enzymes, and structural proteins. Some of the key proteins in each of these categories will be described below.
Sarcomeric Proteins
The sarcomere is the fundamental contractile structure. Sarcomeres are placed end-to-end along the length of each myofibril. A sarcomere is the structure from one Z-disk to the next one, including thick and thin filaments, nebulin, titin, and M-band proteins.
Thick Filaments
The thick filaments are suspended in the middle of the sarcomere and normally situated equidistance from the Z-disks at ether end. The thick filament is mainly composed of myosin and long thin protein strands of titin that are continuous with the thick filament and extend from the ends of the thick filament to the Z-disks on either side.
Myosin the Molecular Motor
Myosin is a superfamily of motor proteins, serving several functions in living creatures. Myosin 2 is the class of myosin that serves the contractile function in skeletal muscle. Each myosin molecule is composed of heavy meromyosin and light meromyosin. The light meromyosin comprises much of the long strand of myosin that forms the thick filament. Heavy meromyosin constitutes the S1 and S2 segments of myosin. Each myosin molecule is formed by two identical halves with the light meromyosin wound together in a helix and the heavy meromyosin free from the thick filament. In mammalian and amphibian muscles, the thick filaments are 1.6 m long (see Figure 4-15). Half of the myosin molecules are oriented in one direction and half are in the other direction. The S2 segment is about 40 nm long and the S1 segment, which contains the actin-binding site and the nucleotide binding site is about 19 nm long.
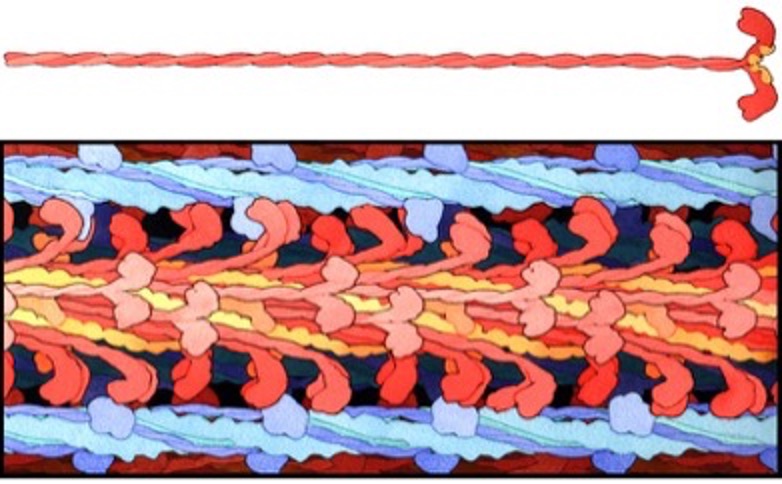
Myosin S1 head
Each S1 head (see Figure 4-16) has an essential light chain and a regulatory light chain (also known as myosin light chain or MLC). The essential light chains interact with actin, facilitating ATPase activity (Sitbon et al. 2019). The regulatory light chains can be phosphorylated MLC-kinase and this phosphorylation results in increased Ca2+ sensitivity (Sweeney et al. 1993). Further discussion of this property is presented in Chapter 13.
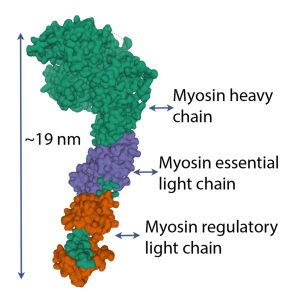
Titin
Titin (previously called connectin) is very large protein, with lengths greater than 1 μm in human skeletal muscle, that is an integral part of the thick filament structure. Titin is the third most abundant protein in skeletal muscle; actin and myosin are more abundant (by mass). As titin connects the z-disk to the m-line in the sarcomere, forming the core of the thick filament (See Figure 4-17). Six strands of titin are associated with every thick myosin filament. The 6 titin strands that protrude from the myosin are each connected to one of the 6 surrounding thin filaments at the z-disk.
It has long been recognized that titin contributes to the passive force, that is the force maintained by the structure of the passive muscle, as opposed to the force created by a metabolic process such as actin-myosin–ATPase, during muscle (Joumaa et al. 2008; Maruyama et al. 1981). In this role it is considered to work like a passive spring. There is a growing amount of evidence that titin can and will change stiffness when the PVEK regions interact with calcium and increases the number of folds in the protein in those sections changing the passive force during subsequent stretch (DuVall et al. 2013). More recently, titin has been proposed as playing a role in residual force enhancement (Herzog 2018), although there is still much debate about the exact mechanism of the process.
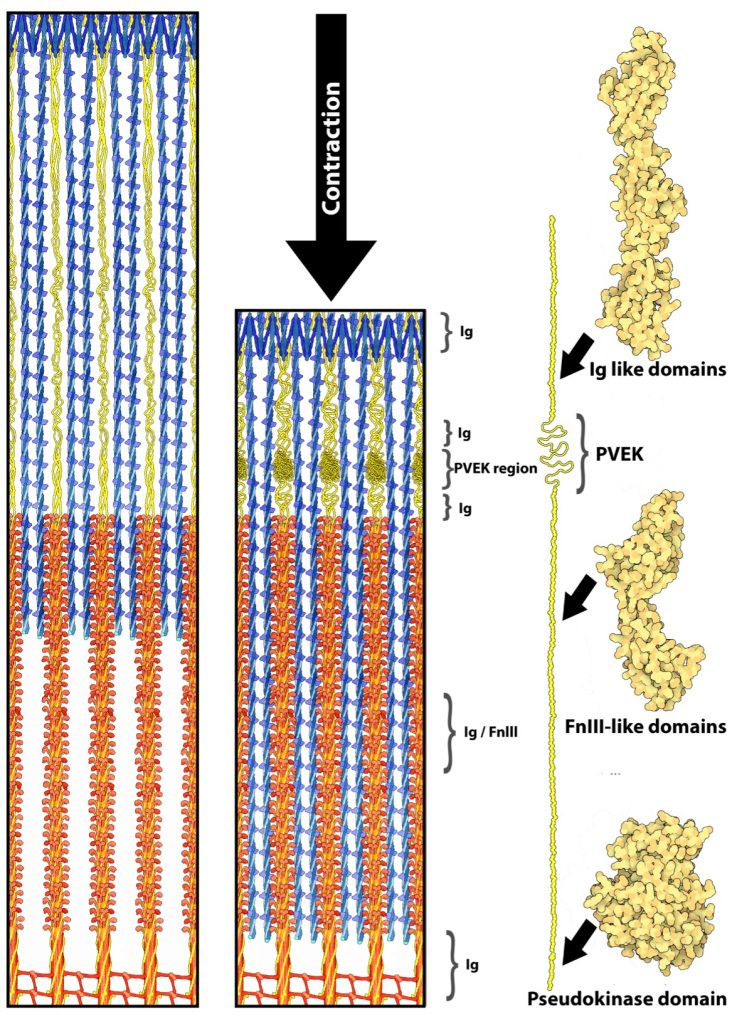
Thin Filaments
The thin filaments are composed of actin and nebulin and contain the regulatory proteins: troponin and tropomyosin. Thin filaments vary in length from 1 μm in amphibian skeletal muscle to 1.3 μm in human skeletal muscle. The length of the thin filament affects the relationship between sarcomere length and active force generated by the muscle (see Chapter 5). Thin filaments also have the regulatory proteins, troponin and tropomyosin associated with them. Each tropomyosin spans 7 actin monomers and has one troponin complex associated with it. The troponin complex is comprised of troponin I, troponin C and troponin T. Troponin C is the subunit that binds Ca2+ to initiate contraction.
Actin
Globular or G-actin is a small protein that polymerizes to form microfilaments. Each G-actin is about 6 nm in diameter (see Figure 4-18). The microfilaments of actin are important for two purposes: i) primary protein of the thin filaments in sarcomeres of muscle and ii) they form part of the cytoskeleton, contributing to the structure of the myocyte.
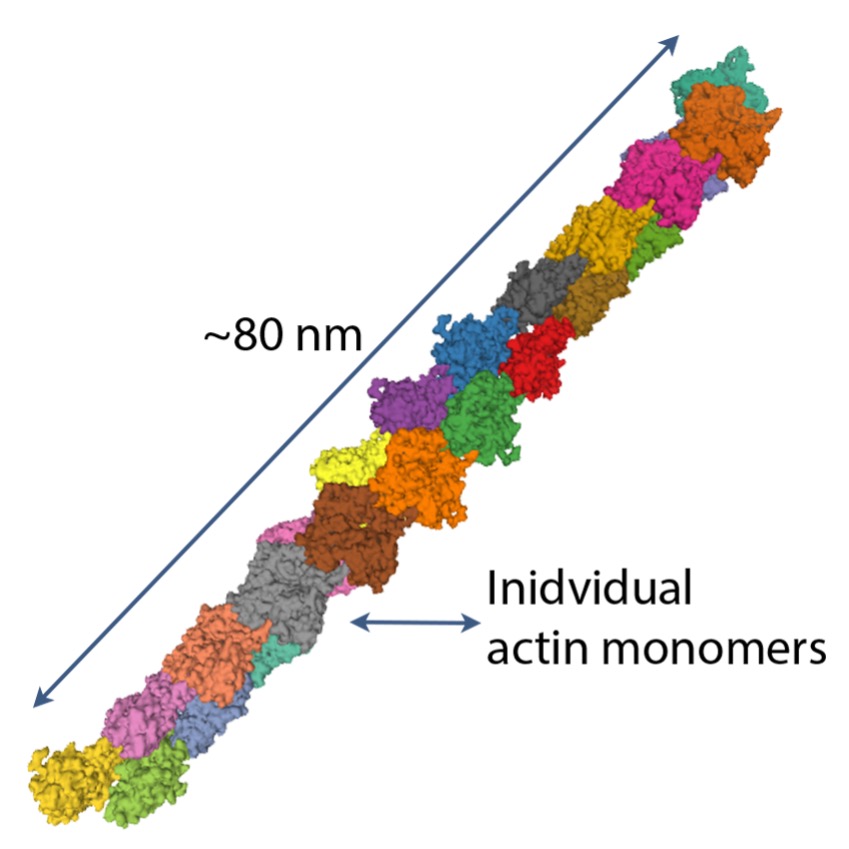
Nebulin
Nebulin is a filamentous protein that binds to actin as it winds along the thin filament. It is not known for sure what the exact roles of nebulin are in the sarcomere, but nebulin has been proposed to play a role in guiding the synthesis of the thin filament.
Regulatory Proteins, Troponin and Tropomyosin
Without the regulatory proteins, troponin and tropomyosin, actin–myosin interaction would proceed randomly, without control. Muscles would always be contracting, and energy needs would be extremely high. This type of energy demand actually occurs during an episode of malignant hyperthermia, a situation where Ca2+ is spontaneously released from the sarcoplasmic reticulum in response to a volatile anesthetic, halothane. Halothane is no longer used in the operating room, because the heat generated from activated muscles of sensitive individuals was lethal.
Tropomyosin is a short filamentous protein that lies in the groove of the helix of f-actin, spanning a segment that is 7 actin monomers long (see Figure 4-19). When tropomyosin is lying in the groove in this way, it obstructs the interaction of the S1 head of myosin with actin. The troponin complex binds to tropomyosin and to actin.
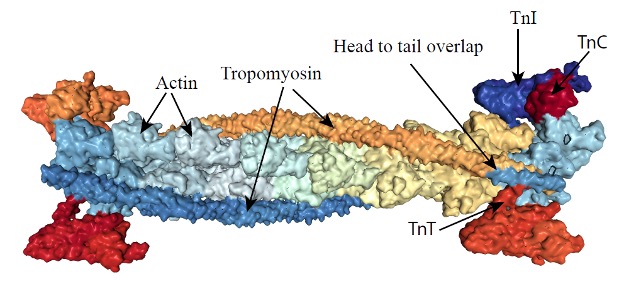
Troponin is a complex of 3 smaller proteins. Troponin-T binds troponin to actin. Troponin-I combine tropomyosin filaments and hold them in the groove of the actin, inhibiting interaction of actin with myosin. Troponin-C is a calcium-binding protein, similar to calmodulin. When Ca2+ binds to troponin–C, the arrangement of the three troponins will change, resulting in the movement of tropomyosin out of the groove between the actin strands, revealing the myosin binding sites on the exposed actin molecules. This interaction and the consequences of it are further described in Chapter 5.
Enzymes
Enzymes. Catalyze reactions, allowing the reactions to proceed much faster than would otherwise be the case. There are several categories of enzymes that are prevalent in skeletal muscle including: kinases, synthases, dehydrogenases, and phosphatases. Some of these will be dealt with later in the appropriate chapters.
Receptors
Receptor proteins are embedded in lipid bilayer membranes. In skeletal muscle, membranes that contain receptor proteins include: plasmalemma, mitochondria, and sarcoplasmic reticulum. One of the major functions of receptor proteins is the transfer of information. For example, hormones will bind to their specific receptor proteins to effect a change in the target cell. Several hormones have specific binding proteins in the sarcolemma: insulin, epinephrine, thyroxin, testosterone, estrogen, growth hormone and others. In each case, a specific protein receptor is embedded in the membrane and this receptor may be linked to a series of additional proteins that function to translate the signal of a change in hormone concentration.
Channels and Pumps
Skeletal muscle membranes contain a number of channels and pumps (transporters). Channels allow the movement of ions down their concentration gradient and transporters move substances across the membrane with or against a concentration gradient. Channels have special structural features including gates, pores and binding sites. Gates allow opening and closing of the pore. The pore is an open space that allows movement of specific ions through the membrane. Restricting the passage of other ions is accomplished by a series of charged amino acids that are referred to as the selectivity filter. Some examples of channels and pumps are presented below. In addition to these channels and pumps, there are several additional transport proteins embedded in the membranes of the myocyte.
Voltage dependent Sodium Channel
The voltage dependent sodium channel in the sarcolemma allows Na+ to move down it’s concentration gradient into the myocyte during activation. This channel is activated by a depolarization of the sarcolemma to about -50 mV and closes when membrane potential depolarizes to +30 mV. This channel is somewhat unique in that it contains two gates (parts of the molecule that obstruct the pore). One of these gates is open in the resting state and the other one is closed. The closed one will open on depolarization to -50mV and the other will close to inactivate the channel at +30mV. Resetting to a state that can be activated requires closure of the open gate and opening of the closed one.
Voltage Dependent Potassium Channel
The voltage dependent potassium channel is just as important as the voltage dependent sodium channel. The voltage dependent potassium channel opens at a similar membrane voltage as the voltage dependent sodium channel, but movement of K+ through the channel out of the myocyte is initially very slow because the electrochemical gradient does not favour movement of K+. Once the action potential causes depolarization, and the Na+ channel closes, the movement of K+ out of the myocyte will repolarize the membrane. This channel closes when the membrane potential reaches -70 mV.
Potassium-ATP Channel
The potassium ATP channel (KATP) is a unique channel that may be involved in regulating activation of muscle when energy charge is challenged (MacIntosh et al. 2012). This K+ channel is regulated by [ATP], making it a sensor of the energy charge in skeletal muscle. Binding of ATP to the channel will close the channel, so when [ATP] falls, resulting in less binding, the KATP channel will open. Opening the channel makes the membrane more permeable to K+. This increased permeability will cause the action potential to be of smaller magnitude, resulting in a smaller magnitude of activation (less Ca2+ release).
Dihydropyridine Receptor (Cav1.1)
The skeletal muscle dihydropyridine dihydropyridine receptor (DHPR) is a specialized Ca2+ channel that exists at high density in the transverse-tubules where they are linked to ryanodine receptors (See Figure 4-14). The movement of Ca2+ through these channels is not necessary for activation of the myocyte. This is because the DHPR detects a voltage change in the plasmalemma and opens the ryanodine in response.
Ryanodine Receptor
The ryanodine ryanodine receptor (RYR) gets its name from the fact that ryanodine binds to this receptor at very low concentration, locking the receptor in the open configuration. The RYR are very large protein channels (> 2.2 MDa) that are found in the terminal cisternae of the SR (see Figure 4-14). The RYR are formed through the interlocking of four repeating protein structures (see Figure 4-20). The resulting physical channel formed by the four interlocking proteins opens and closes to allow or prevent the passage of Ca2+ based on the structural arrangement of the four proteins. The RYR is opened during the action potential by direct mechanical link to the DHPR. The RYR remains open very briefly as a high cytoplasmic [Ca2+] causes the channel to close. There are three isoforms of the RYR found in the human body with RYR 1 being the isoform found in skeletal muscle. Every second RYR 1 is connected to a voltage sensitive DHPR embedded in the in the membrane of the adjacent transverse-tubule (See Figure 4-15) (Nelson et al. 2013).
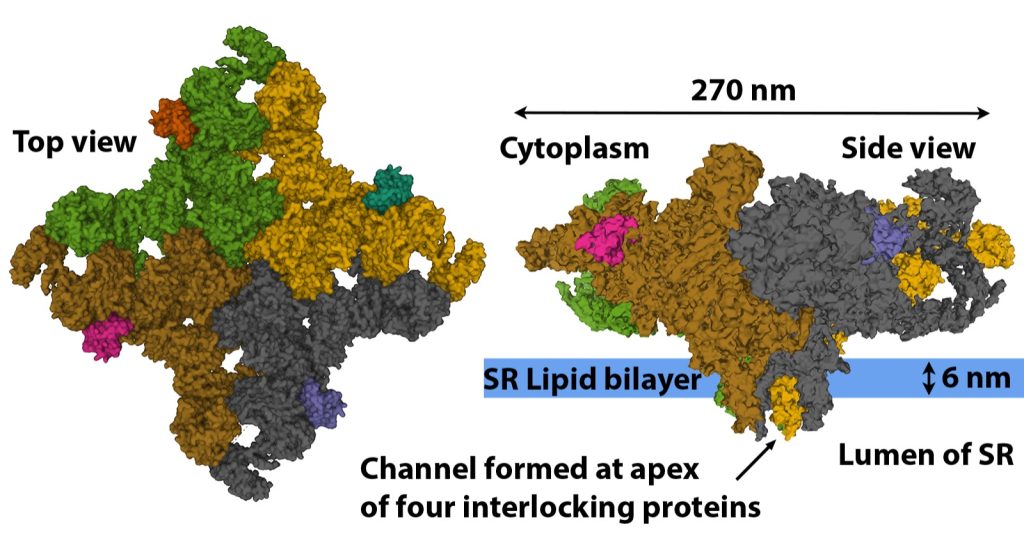
Sodium-Potassium ATPase
The sodium potassium ATPase (Na+–K+ATPase) is an important ion pump in the sarcolemma of both neurons and myocytes. These cells allow communication by propagation of action potential during which there is a loss of cellular K+ and accumulation of intracellular Na+. Recovery to the normal resting state is accomplished in part by the action of these ion pumps.
Sarcoendoplasmic reticulum calcium ATPase (SERCA)
Following activation of a myocyte, when Ca2+ has been released into the cytoplasm, it must be returned to the sarcoplasmic reticulum to allow relaxation. This transport of Ca2+ out of the cytoplasm is accomplished by a Ca2+ATPase known as SERCA. This transporter moves 2 ions of Ca2+ for each ATP used. It has been estimated that the energy cost of transporting Ca2+ back into the SR can account for about 30% of the energy cost of an isometric muscle contraction.
Human skeletal muscles contain two isoforms of the SERCA protein, which are uniquely associated with each muscle fibre type. Somewhat confusingly SERCA1 proteins are associated with type II muscle fibres whereas SERCA2 proteins are associated with Type I muscle fibres. While the different isoforms of SERCA function in nearly identical fashion, pumping 2 Ca2+ across the membrane for every molecule of ATP used, their calcium sensitivity, transport speed, and density in the SR are distinct between the two isoforms.
The density and sensitivity of the two isoforms of SERCA are aligned with the activity and morphology of the types of muscle fibres they are associated with. The SERCA2 in the slower type I fibres are more sensitive to calcium concentration and reach their peak activity at lower [Ca2+] (~pCa 6.8) than SERCA1 in the faster type II fibres (pCa 6.6) (Lamboley et al. 2014). Also, within type II fibres, the density of SERCA1 is more than double that of the SERCA2 in the type I muscle fibres (Lombardi et al 2014).
SERCA pumps are effective in maintaining a very low resting [Ca2+] of ~50nM and reducing [Ca2+] after muscular activation. Lombardi et al. 2014 calculated that human SERCA from the vastus lateralis muscle have a maximum Ca2+ removal rates of roughly 0.9 mmol per litre per second, which would allow the muscle to completely relax in less than 0.25 of a second. It should be noted though that estimates of SERCA rates in exclusively IIx fibres are substantially higher.
SERCA contributes to the use of ATP during muscle contraction—
The required abundance and density of SERCA proteins in the SR combined with their high activity rates make the SERCA pumps a significant energy consumer during muscle contraction. This means SERCA pumps are a main contributor to heat production during muscle activity. Even at rest SERCA pumps within the muscle are actively pumping Ca2+ out of the cytosol and back into the SR to combat leak of Ca2+ from the SR. While this leak rate is low in humans it was measured as ~6 and ~4 µmol per litre per second in human type I and type II fibres (Lombardi et al 2014). The continual activity of the SERCA pumps is thought to help contribute to the maintenance of body temperature.
Summary
Our muscles give our body shape and the structures of muscle allow the many functions to be achieved. Muscles can be long or short, wide or narrow. Muscles are composed of connective tissue and myocytes. The myocyte generate force and shorten and the connective tissue allows transfer of force laterally and to the bones of our skeleton. Muscle cells are organized either in line with the action of the muscle (parallel fibred, or fusiform) or at some angle relative to the line of action (pinnate). Muscle cells (also called fibres or myocytes) are organized into units referred to as motor units where each motor unit is a single motoneuron and all of the myocytes that cell innervates. Motor units can be small with just a few myocytes, or very large with thousands of myocytes. Individual myocytes have the common parts that all cells of the body have: nucleus (several per cell), mitochondria, cytoskeleton, ribosomes and endoplasmic reticulum. Unique to muscle cells, they have myofibrils, or tubes composed of sarcomeres, repeating structures containing the contractile proteins, actin and myosin. The neuromuscular junction serves the important purpose of translating an activation signal from the motoneuron to activation of the myocyte. The neuromuscular junction includes the nerve terminal, and the end-plate of the myocyte as well as the gap between these two. The endplate is folded allowing increased membrane surface area, where large numbers of acetylcholine receptors are embedded. Muscles also have specialized sensors that serve to inform the central nervous system of the length, force and metabolic circumstances at any given time. The most elaborate of these sensors is the spindle which is composed of several intrafusal muscle fibres and has both afferent and efferent innervation. Muscles also have several proteins, many of which are unique to contractile tissue. Generally, proteins can be categorized as: channels, pumps, receptors, enzymes, binding proteins and structural proteins. Additionally, muscles have motor proteins to allow them to generate force and movement.
Highlight Features- Research
For this research highlight, we turn to a classic paper, published in the early 70’s. Burke and colleagues (Burke, 1973) experimented on anesthetized cats to investigate the contractile properties of motor units and established the nomenclature for assessment of fibre-types. They isolated the nerve of the gastrocnemius muscle and stimulated individual axons with an intracellular electrode. They discovered that the contractile properties of the motor units could be classified as three fibre-types: slow-twitch (S), fast-twitch fatigue resistant (FFR) and fast-twitch fatiguable (FF). Two properties in particular were consistent and valuable in identifying these fibre-types. The first of these properties was sag. When FF and FFR units were stimulated for 1 s with trains of pulses at subfusion frequencies, eliciting an incompletely fused tetanic contraction, they observed sag. Force would reach a peak early in the stimulation, then decrease, or sag, before the stimulation stopped. This sag or decrease in force was not evident when S motor units were stimulated in this way. The authors then stimulated the axon with a 330 ms train of pulses at 40 Hz, repeated every second. Some units (FF) decreased in force very quickly while other units (FFR and S) were slow to decline in force. A fatigue index was calculated. Fatigue index was calculated as the end active force divided by the initial active force. It was found that when the fatigue index was less than 0.25, motor units were FF. The fatigue index of FFR units was above 0.75. S units had a fatigue index of 0.9 or greater. When the axon of a motor unit was stimulated with a single pulse, eliciting a twitch, slow-twitch motor units had a long time to peak, and fast-twitch motor units had a short time to peak. However, this feature was not as useful as the properties described above for separating the 3 classifications of fibre-type. Note that a small percentage of units could not be classified with the fatigue index and presence of sag. It could be that these difficult units were hybrid motor units.
For some units, histochemical evaluation was completed. First, these units were stimulated for a long time to deplete the glycogen. Then the muscle was quick frozen to preserve it. Then, sequential slices were obtained, so individual fibres could be identified in sequential slices. Slices of the muscle were chemically treated to “stain” under certain circumstances. First, these slices were used to identify the individual fibres of the stimulated motor unit. This was done by staining for glycogen. The fibres belonging to the stimulated motor unit had very little glycogen, so they stained very pale in comparison to unstimulated fibres. The authors also performed histological assessment for the presence of enzymes in the aerobic pathways (succinyl dehydrogenase) and anaerobic pathway (lactate dehydrogenase). When staining for succinyl dehydrogenase the darkest fibres were in the S units and FFR units were almost as dark, while this stain was pale in the FF units. When staining for lactate dehydrogenase, the darkest fibres were FF units and FFR units were almost as dark. S units stained less. This research article includes additional contractile and histochemical properties of motor units of the cat gastrocnemius muscle. Read the article to find out more about these fibre-type properties.
References
Alway, S.E. 1991. Is fiber mitochondrial volume density a good indicator of muscle fatigability to isometric exercise? Journal of Applied Physiology 70(5): 2111-2119.
Alway, S.E., MacDougall, J.D., Sale, D.G., Sutton, J.R., and McComas, A.J. 1988. Functional and structural adaptations in skeletal muscle of trained athletes. Journal of Applied Physiology 64(3): 1114-1120. Available from PM:3366734 [accessed -32676///].
Axelsson, J., and Thesleff, S. 1959. A study of supersensitivity in denervated mammalian skeletal muscle. Journal of Physiology 147: 178-193.
Brown, I.E., Kim, D.H., and Loeb, G.E. 1998. The effect of sarcomere length on triad location in intact feline caudofeomoralis muscle fibres 1. J.Muscle Res.Cell Motil. 19(5): 473-477. Available from PM:9682134
http://www.ingentaconnect.com/content/klu/jure/1998/00000019/00000005/00176802?token=004f1ab3c18c285459383a4b3b25702a7b427a247b422c7463662a726e2d2954496f642f466fe3b [accessed.
Burke, R.E., Levine, D.N., Tsairis, P., and Zajac, F.E. 1973. Physiological types and histochemical profiles in motor units of the cat gastrocnemius. Journal of Physiology 234: 723-748.
des Georges, A., Clarke, O.B., Zalk, R., Yuan, Q., Condon, K.J., Grassucci, R.A., et al. 2016. Structural Basis for Gating and Activation of RyR1. Cell 167(1): 145-157.e117. doi:10.1016/j.cell.2016.08.075.
DuVall, M.M., Gifford, J.L., Amrein, M., and Herzog, W. 2013. Altered mechanical properties of titin immunoglobulin domain 27 in the presence of calcium. European Biophysics Journal 42(4): 301-307. doi:10.1007/s00249-012-0875-8.
Fortuna, R., Aurélio Vaz, M., Rehan Youssef, A., Longino, D., and Herzog, W. 2011. Changes in contractile properties of muscles receiving repeat injections of botulinum toxin (Botox). Journal of Biomechanics 44(1): 39-44. doi:https://doi.org/10.1016/j.jbiomech.2010.08.020.
Francis, N., Cohen-Solal, A., and Logeart, D. 1999. Peripheral muscle ergoreceptors and ventilatory response during exercise recovery in heart failure. American Journal of Physiology-Heart and Circulatory Physiology 276(3): H913-H917. doi:10.1152/ajpheart.1999.276.3.H913.
Herzog, W. 2018. The multiple roles of titin in muscle contraction and force production. Biophys Rev 10(4): 1187-1199. doi:10.1007/s12551-017-0395-y.
Hill, A.V. 1953. The mechanics of active muscle. Proceedings of the Royal Society of London.Series B: Biological Sciences 141: 104-117.
Himmel, D.M., Gourinath, S., Reshetnikova, L., Shen, Y., Szent-Györgyi, A.G., and Cohen, C. 2002. Crystallographic findings on the internally uncoupled and near-rigor states of myosin: Further insights into the mechanics of the motor. Proceedings of the National Academy of Sciences 99(20): 12645-12650. doi:10.1073/pnas.202476799.
Janssen, I., Heymsfield, S.B., Wang, Z., and Ross, R. 2000. Skeletal muscle mass and distribution in 468 men and women aged 18–88 yr. Journal of Applied Physiology 89(1): 81-88. doi:10.1152/jappl.2000.89.1.81.
Joumaa, V., Leonard, T.R., and Herzog, W. 2008. Residual force enhancement in myofibrils and sarcomeres. Proceedings of the Royal Society B: Biological Sciences 275(1641): 1411-1419. doi:10.1098/rspb.2008.0142.
Lamboley, C.R., Murphy, R.M., McKenna, M.J., and Lamb, G.D. 2014. Sarcoplasmic reticulum Ca2+ uptake and leak properties, and SERCA isoform expression, in type I and type II fibres of human skeletal muscle. The Journal of Physiology 592(6): 1381-1395. doi:https://doi.org/10.1113/jphysiol.2013.269373.
Landstrom, A.P., Beavers, D.L., and Wehrens, X.H.T. 2014. The junctophilin family of proteins: from bench to bedside. Trends in Molecular Medicine 20(6): 353-362. doi:10.1016/j.molmed.2014.02.004.
Levine, R.J.C., Kensler, R.W., Yang, Z.H., and Sweeney, H.L. 1995. Myosin regulatory light chain phosphorylation and the production of functionally significant changes in myosin head arrangement on striated muscle thick filaments. Biophysical Journal 68 Suppl.: 224S-224S.
MacDougall, J.D., Sale, D.G., Alway, S.E., and Sutton, J.R. 1984. Muscle fiber number in biceps brachii in bodybuilders and control subjects. Journal of Applied Physiology 57(5): 1399-1403. Available from PM:6520032 [accessed.
MacIntosh, B.R., Gardiner, P.F., and McComas, A.J. 2006. Skeletal Muscle: Form and Function, 2nd Edition. Human Kinetics Publishers, Champaign, ILL.
MacIntosh, B.R., Holash, R.J., and Renaud, J.-M. 2012. Control of skeletal muscle contraction: muscle fatigue is a regulated response. J Cell Sci 125: 2105-2114.
Maruyama, K., Kimura, S., Ohashi, K., and Kuwano, Y. 1981. Connectin, an Elastic Protein of Muscle. Identification of “Titin” with Connectin 1. The Journal of Biochemistry 89(3): 701-709. doi:10.1093/oxfordjournals.jbchem.a133249.
McNeil, C.J., Doherty, T.J., Stashuk, D.W., and Rice, C.L. 2005. Motor unit number estimates in the tibialis anterior muscle of young, old, and very old men. Muscle & Nerve 31(4): 461-467. doi:10.1002/mus.20276.
Monti, R.J., Roy, R.R., Hodgson, J.A., and Edgerton, V.R. 1999. Transmission of forces within mammalian skeletal muscles. Journal of Biomechanics 32(4): 371-380. Available from PM:10213027 [accessed.
Murphy, R.M., Dutka, T.L., Horvath, D., Bell, J.R., Delbridge, L.M., and Lamb, G.D. 2013. Ca2+-dependent proteolysis of junctophilin-1 and junctophilin-2 in skeletal and cardiac muscle. The Journal of Physiology 591(3): 719-729. doi:https://doi.org/10.1113/jphysiol.2012.243279.
Nelson, B.R., Wu, F., Liu, Y., Anderson, D.M., Mcanally, J., Lin, W., et al. 2013. Skeletal muscle-specific T-tubule protein STAC3 mediates voltage-induced Ca2+ release and contractility. PNAS 110(29): 11881-11886. doi:10.1073/pnas.1310571110.
Paul, D.M., Squire, J.M., and Morris, E.P. 2017. Relaxed and active thin filament structures; a new structural basis for the regulatory mechanism. Journal of Structural Biology 197(3): 365-371. doi:10.1016/j.jsb.2017.01.004.
Persechini, A., Stull, J.T., and Cooke, R. 1985. The effect of myosin phosphorylation on the contractile properties of skinned rabbit skeletal muscle fibers. Journal of Biological Chemistry 260(13): 7951-7954.
Pfanner, N., Warscheid, B., and Wiedemann, N. 2019. Mitochondrial proteins: from biogenesis to functional networks. Nature Reviews Molecular Cell Biology 20(5): 267-284. doi:10.1038/s41580-018-0092-0.
Piepoli, M., Clark, A.L., and Coats, A.J.S. 1995. Muscle metaboreceptors in hemodynamic, autonomic, and ventilatory responses to exercise in men. American Journal of Physiology: Heart Circulatory Physiology 269(4 Pt 2): H1428-H1436. Available from PM:7485577 [accessed.
Ross, J.A., Levy, Y., Svensson, K., Philp, A., Schenk, S., and Ochala, J. 2018. SIRT1 regulates nuclear number and domain size in skeletal muscle fibers. Journal of cellular physiology 233(9): 7157-7163. doi:10.1002/jcp.26542.
Sanchez, E.J., Lewis, K.M., Danna, B.R., and Kang, C. 2012. High-capacity Ca<sup>2+</sup> Binding of Human Skeletal Calsequestrin *<sup></sup>. Journal of Biological Chemistry 287(14): 11592-11601. doi:10.1074/jbc.M111.335075.
Sehnal, D., Bittrich, S., Deshpande, M., Svobodová, R., Berka, K., Bazgier, V., et al. 2021. Mol* Viewer: modern web app for 3D visualization and analysis of large biomolecular structures. Nucleic Acids Research 49(W1): W431-W437. doi:10.1093/nar/gkab314.
Shishmarev, D. 2020. Excitation-contraction coupling in skeletal muscle: recent progress and unanswered questions. Biophys Rev 12(1): 143-153. doi:10.1007/s12551-020-00610-x.
Sitbon, Y.H., Yadav, S., Kazmierczak, K., and Szczesna‐Cordary, D. 2019. Insights into myosin regulatory and essential light chains: a focus on their roles in cardiac and skeletal muscle function, development and disease. Journal of Muscle Research and Cell Motility. doi:10.1007/s10974-019-09517-x.
Sweeney, H.L., Bowman, B.F., and Stull, J.T. 1993. Myosin light chain phosphorylation in vertebrate striated muscle: Regulation and function. American Journal Physiology – Cell Physiology 264(5 Pt 1): C1085-C1095. Available from PM:8388631 [accessed.
Termin, A., Staron, R.S., and Pette, D. 1989. Myosin heavy chain isoforms in histochemically defined fiber types of rat muscle. Histochemistry 92(6): 453-457. doi:10.1007/BF00524756.
Windhorst, U. 2007. Muscle proprioceptive feedback and spinal networks. Brain Research Bulletin 73(4-6): 155-202. doi:10.1016/j.brainresbull.2007.03.010.
Yaraskavitch, M., Leonard, T., and Herzog, W. 2008. Botox produces functional weakness in non-injected muscles adjacent to the target muscle. Journal of Biomechanics 41(4): 897-902. doi:https://doi.org/10.1016/j.jbiomech.2007.11.016.
Meet the Authors
Brian R. MacIntosh, Faculty of Kinesiology, University of Calgary, Calgary, Alberta, Canada
Dr. Brian MacIntosh obtained his undergraduate degree in Human Kinetics at the University of Guelph in 1975. He completed his PhD in 1979 at the University of Florida in Medical Sciences under the supervision of Dr. Wendell Stainsby. After a brief postdoctoral fellowship at Queens University in the Dept of Physiology, he held faculty positions at Concordia University (1980-1986), University of Saskatchewan (1986-1989) and the University of Calgary (1989-2019). He is now retired, but still pursuing research as Professor Emeritus. During his career, Dr. MacIntosh published articles on a variety of topics: skeletal muscle fatigue, activity dependent potentiation, atrophy, running economy, force-velocity properties and power output.
R. John Holash, PhD, Faculty of Kinesiology, University of Calgary, Calgary, Alberta, Canada
Dr. John Holash obtained his undergraduate degree in Physical Education at the University of Calgary in 1994. He then worked as an outdoor guide before returning to the University of Calgary to obtain his Master of Science in Exercise Physiology and Biomechanics in 2000. John then worked professionally for the University of Calgary as the head of the computer technology department in the Faculty of Kinesiology (1997-2019). John completed his PhD in 2017 at the University of Calgary and currently holds a faculty position at the University of Calgary, in the Faculty of Kinesiology. His current research topics include computational modeling of skeletal and cardiac muscle, skeletal muscle structure and function, exergaming, and wearable fitness technologies. John is a competitive master’s athlete in biathlon, triathlon, and cycling.
blood vessel of the microcirculation. Exchange of gas (O2 and CO2) as well a small molecules and water occurs across capillary walls